Enzyme: Difference between revisions
TimVickers (talk | contribs) No edit summary |
(3ucky(3all (talk | contribs) m partial rv. of last edit by TimVickers: enzymes very regularly change conformation during a reaction. |
||
Line 5: | Line 5: | ||
Enzymes are essential to life because most chemical reactions in living cells would occur too slowly, or would lead to different products without enzymes. |
Enzymes are essential to life because most chemical reactions in living cells would occur too slowly, or would lead to different products without enzymes. |
||
Like all catalysts, enzymes work by providing an alternate pathway of lower activation energy to a reaction, thus allowing the reaction to proceed much faster. Enzymes may speed up reactions by a factor of many millions. An enzyme, like any catalyst, remains unaltered |
Like all catalysts, enzymes work by providing an alternate pathway of lower activation energy to a reaction, thus allowing the reaction to proceed much faster. Enzymes may speed up reactions by a factor of many millions. An enzyme, like any catalyst, remains unaltered by the completed reaction and can therefore function repeatedly. Because enzymes do not affect the relative energy between the products and reagents, they do not affect the [[Chemical equilibrium|equilibrium]] of a reaction. However, the main advantage of enzymes over most other catalysts is their very high specificity. |
||
Enzyme activity can be affected by other [[molecules]]. [[Enzyme inhibitor|Inhibitors]] are naturally occurring or synthetic molecules that decrease or abolish enzyme activity; while activators are molecules that increase activity. Some irreversible inhibitors bind enzymes very tightly, effectively inactivating them. |
Enzyme activity can be affected by other [[molecules]]. [[Enzyme inhibitor|Inhibitors]] are naturally occurring or synthetic molecules that decrease or abolish enzyme activity; while activators are molecules that increase activity. Some irreversible inhibitors bind enzymes very tightly, effectively inactivating them. |
Revision as of 15:10, 28 June 2006
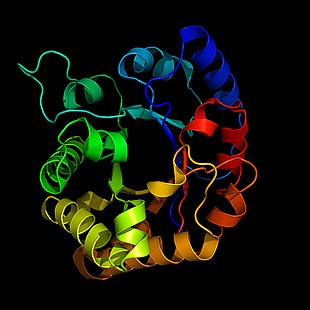
An enzyme is a macromolecule that catalyzes a chemical reaction. Most enzymes are proteins and the word "enzyme" is often used to mean a protein enzyme, but some RNA molecules also have catalytic activity, and to differentiate them from protein enzymes, they are referred to as RNA enzymes or ribozymes.
Enzymes are essential to life because most chemical reactions in living cells would occur too slowly, or would lead to different products without enzymes.
Like all catalysts, enzymes work by providing an alternate pathway of lower activation energy to a reaction, thus allowing the reaction to proceed much faster. Enzymes may speed up reactions by a factor of many millions. An enzyme, like any catalyst, remains unaltered by the completed reaction and can therefore function repeatedly. Because enzymes do not affect the relative energy between the products and reagents, they do not affect the equilibrium of a reaction. However, the main advantage of enzymes over most other catalysts is their very high specificity.
Enzyme activity can be affected by other molecules. Inhibitors are naturally occurring or synthetic molecules that decrease or abolish enzyme activity; while activators are molecules that increase activity. Some irreversible inhibitors bind enzymes very tightly, effectively inactivating them.
While all enzymes have a biological role, some enzymes are used commercially. Many household cleaners use enzymes to speed up chemical reactions ( e.g., breaking down protein or starch stains in clothes).
More than 5,000 enzymes are known. Typically the suffix -ase is added to the name of the substrate (e.g., lactase is the enzyme that catalyzes the cleavage of lactose) or the type of reaction (e.g., DNA polymerase catalyzes the formation of DNA polymers). However, this is not always the case, especially when enzymes modify multiple substrates. For this reason Enzyme Commission or EC numbers are used to classify enzymes based on the reactions they catalyze. Even this is not a perfect solution, as enzymes from different species or even very similar enzymes in the same species may have identical EC numbers.
Etymology and history

The word enzyme comes from Greek: "in leaven". As early as the late 1700s and early 1800s, the digestion of meat by stomach secretions and the conversion of starch to sugars by plant extracts and saliva were known. However, the machanism by which this occured was unknown.[1]
In the 19th century, when studying the fermentation of sugar to alcohol by yeast, Louis Pasteur came to the conclusion that this fermentation was catalyzed by a vital force contained within the yeast cells called "ferments", which were thought to function only within a living organisms. He wrote that "Alcoholic fermentation is an act correlated with the life and organisation of the yeast cells, not with the death or putrefaction of the cells."[2]
In 1897, Eduard Buchner studied the ability of yeast extracts to ferment sugar, despite the absence of living yeast cells. In a series of experiments at the University of Berlin he found that the sugar was fermented, even when there were no living yeast cells in the mixture [3]. He named the enzyme in this extract that brought about the fermentation of sucrose "zymase". [4].
It was not until 1926, however, that pepsin became the first enzyme to be obtained in pure form by Northrop, Sumner and Stanley. Their successful purification and crystallization of pepsin proved that enzymes were proteins and they were awarded the 1946 Nobel prize for Chemistry [5].
Enzyme structure and mechanism
In enzymes, as with other proteins, function is determined by structure. An enzyme can be:
- A monomeric protein, i.e., containing only one polypeptide chain, typically one hundred or more amino acids; or
- An oligomeric protein consisting of several polypeptide chains, different or identical, that act together as a unit.
As with any protein, each monomer is actually produced as a long, linear chain of amino acids, which folds in a particular fashion to produce a three-dimensional product. Individual monomers may then combine via non-covalent interactions to form a multimeric protein. Many enzymes can be unfolded or inactivated by heating, which destroys the three-dimensional structure of the protein.
Most enzymes are larger than the substrates they act on and only a very small portion of the enzyme, around 10 amino acids, comes into direct contact with the substrate(s). This region, where binding of the substrate(s) and then the reaction occurs, is known as the active site of the enzyme. Some enzymes contain sites that bind cofactors, which are needed for catalysis. Certain enzymes have binding sites for small molecules, which are often direct or indirect products or substrates of the reaction catalyzed. This binding can serve to increase or decrease the enzyme's activity (depending on the molecule and enzyme), providing a means for feedback regulation.
Specificity
Enzymes are usually specific as to the reactions they catalyze and the substrates that are involved in these reactions. Shape, charge complementarity, and hydrophilic/hydrophobic characters of enzymes and substrates are responsible for this specificity. Enzymes show impressive levels of stereospecificity, regioselectivity and chemoselectivity.
"Lock and key" model


Enzymes are very specific and it was suggested by Emil Fischer in the 1890s that this was because the enzyme had a particular shape into which the substrate(s) fit exactly. This is often referred to as "the lock and key" model. An enzyme combines with its substrate(s) to form a short-lived enzyme-substrate complex. However, while this model explains enzyme specificity, it fails to explain the stabilisation of the transition state which occurs.
Induced fit model
In 1958 Daniel Koshland suggested a modification to the "lock and key" model. Enzymes are rather flexible structures. The active site of an enzyme can be modified as the substrate interacts with the enzyme. The amino acids side chains which make up the active site are molded into a precise shape which enables the enzyme to perform its catalytic function. In some cases the substrate molecule changes shape slightly as it enters the active site. Unlike the "Lock and key" model, this model explains enzyme specificity and the stabilisation of the transition state which occurs.
Modifications
Many enzymes contain not only protein but require additional modifications. These modifications are made posttranslationally; that is, after the polypeptide chain has been synthesized. Additional groups can be added onto the polypeptide chain, e.g., phosphorylation or glycosylation of the enzyme.
Another kind of posttranslational modification is the cleavage of the polypeptide chain. Chymotrypsin, a digestive protease, is produced in inactive form as chymotrypsinogen in the pancreas and transported in this form to the stomach where it is activated. This prevents the enzyme from harmful digestion of the pancreas or other tissue. This type of inactive precursor to an enzyme is known as a zymogen.
Enzyme cofactors
Some enzymes do not need any additional components to exhibit full activity. However, others require non-protein molecules to be bound for activity. Cofactors can be either inorganic (e.g., metal ions and Iron-sulfur clusters) or organic compounds, which are also known as coenzymes.
Enzymes that require a cofactor, but do not have one bound are called apoenzymes. An apoenzyme together with its cofactor(s) constitutes a holoenzyme (i.e., the active form). Most cofactors are not covalently bound to an enzyme, but are closely associated. However, some cofactors known as prosthetic groups are covalently bound (e.g., thiamine pyrophosphate in certain enzymes).
Most cofactors are either regenerated or chemically unchanged at the end of the reactions. Many cofactors are vitamin-derivatives and serve as carriers to transfer electrons, atoms, or functional groups from an enzyme to a substrate. Common examples are NAD and NADP, which are involved in electron transfer and coenzyme A, which is involved in the transfer of acetyl groups.
Allosteric modulation
Allosteric enzymes change their structure in response to binding of effectors. Modulation can be direct, where the effector binds directly to binding sites in the enzyme, or indirect, where the effector binds to other proteins or protein subunits that interact with the allosteric enzyme and thus influence catalytic activity.
Thermodynamics

As with all catalysts, all reactions catalyzed by enzymes must be "spontaneous" (containing a net negative Gibbs free energy). In the presence of an enzyme, a reaction runs in the same direction as it would without the enzyme, just more quickly. However, the uncatalyzed, "spontaneous" reaction might lead to different products than the catalyzed reaction. Furthermore, enzymes can couple two or more reactions, so that a thermodynamically favorable reaction can be used to "drive" a thermodynamically unfavorable one. For example, the cleavage of the high-energy compound ATP is often used to drive other, energetically unfavorable chemical reactions.
Enzymes catalyze the forward and backward reactions equally. They do not alter the equilibrium itself, but only the speed at which it is reached. Carbonic anhydrase catalyzes its reaction in either direction depending on the conditions.
Kinetics
In 1913, Leonor Michaelis and Maud Menten proposed a quantitative theory of enzyme kinetics, which is referred to as Michaelis-Menten kinetics. Their work was further developed by G. E. Briggs and J. B. S. Haldane, who derived numerous kinetic equations that are still widely used today.
Enzymes can perform up to several million catalytic reactions per second; to determine the maximum speed of an enzymatic reaction, the substrate concentration is increased until a constant rate of product formation is achieved. This is the maximum velocity (Vmax) of the enzyme. In this state, all enzyme active sites are saturated with substrate. However, Vmax is only one kinetic parameter that biochemists are interested in. The amount of substrate needed to achieve a given rate of reaction is also of interest. This can be expressed by the Michaelis-Menten constant (Km), which is the substrate concentration required for an enzyme to reach one half its maximum velocity. Each enzyme has a characteristic Km for a given substrate.
The efficiency of an enzyme can be expressed in terms of kcat/Km. The quantity kcat, also called the turnover number, incorporates the rate constants for all steps in the reaction, and is the quotient of Vmax and the total enzyme concentration. kcat/Km is a useful quantity for comparing different enzymes against each other, or the same enzyme with different substrates, because it takes both affinity and catalytic ability into consideration. The theoretical maximum for kcat/Km, called the diffusion limit, is about 108 to 109 (M-1 s-1). At this point, every collision of the enzyme with its substrate will result in catalysis and the rate of product formation is not limited by the reaction rate but by the diffusion rate. Enzymes that reach this kcat/Km value are called catalytically perfect or kinetically perfect. Example of such enzymes are triose-phosphate isomerase, carbonic anhydrase, acetylcholinesterase, catalase, fumarase, ß-lactamase, and superoxide dismutase.
Some enzymes operate with kinetics which are faster than diffusion rates, which would seem to be impossible. Several mechanisms have been invoked to explain this phenomenon. Some proteins are believed to accelerate catalysis by drawing their substrate in and preorienting them by using dipolar electric fields. Some invoke a quantum-mechanical tunneling explanation whereby a proton or an electron can tunnel through activation barriers, although for protons tunneling remains somewhat controversial. [6][7]
Inhibition
Enzymes reaction rates can be decreased by variuos types of enzyme inhibitors.
Mechanisms of enzyme inhibitors
Competitive inhibition
In competitive inhibition, the inhibitor binds to the substrate binding site as shown (right part b), thus preventing substrate binding. Malonate is a competitive inhibitor of the enzyme succinate dehydrogenase, which catalyzes the oxidation of succinate to fumarate.
Competitive inhibition causes the Km value to increase, but does not effect Vmax.
Non-competitive inhibition
Non-competitive inhibitors never bind to the active center, but to other parts of the enzyme that can be far away from the substrate binding site, consequently, there is no competition between the substrate and inhibitor for the enzyme. The extent of inhibition depends entirely on the inhibitor concentration and will not be affected by the substrate concentration. This type of inhibition is typically irreversible, meaning that the enzyme will no longer function.
By changing the conformation (the three-dimensional structure) of the enzyme, the inhibitors either disable the ability of the enzyme to bind or turn over its substrate. The enzyme-inhibitor (EI) and enzyme-inhibitor-substrate (EIS) complex have no catalytic activity.
Non-Competitive inhibition causes a decrease in Vmax, but does not change the Km value.
Partially competitive inhibition
The mechanism of partially competitive inhibition is similar to that of non-competitive, except that the EIS-complex has catalytic activity, which may be lower or even higher (partially competitive activation) than that of the enzyme-substrate (ES) complex.
This inhibition typically displays a lower Vmax, but an unaffected Km value.
Uncompetitive inhibition
Uncompetitive inhibition occurs when the inhibitor binds only to the enzyme-substrate complex, not to the free enzyme; the EIS complex is catalytically inactive. This mode of inhibition is rare and causes a decrease in both Vmax and the Km value.
Mixed inhibition
Mixed inhibitors can bind to both the enzyme and the ES complex. It has the properties of both competitive and uncompetitive inhibition.
Both a decrease in Vmax and an increase in the Km value are seen in mixed inhibition.
Uses of enzyme inhibitors
Inhibitors are often used as drugs, but they can also act as poisons. However, the difference between a drug and a poison is usually only a matter of amount, since most drugs are toxic at some level, as Paracelsus wrote "The dose makes the poison." Equally, antibiotics and other anti-infective drugs are just specific poisons that can kill a pathogen but not its host. Examples of inhibitors being used as drugs or poisons include aspirin, which inhibits the COX-1 and COX-2 enzymes that produce the inflammation messenger prostaglandin, thus suppressing pain and inflammation. The poison cyanide combines with the copper prosthetic groups of the enzyme cytochrome c oxidase and blocking cellular respiration.
In many organisms, inhibitors may act as part of a feedback mechanism. If an enzyme produces too much of one substance in the organism, that substance may act as an inhibitor for the enzyme that produces it, causing production of the substance to slow down or stop when there is sufficient amount. This is a form of negative feedback.
Function and control of enzymes in the cell
Metabolic pathways
Several enzymes can work together in a specific order, creating metabolic pathways. In a metabolic pathway, one enzyme takes the product of another enzyme as a substrate. After the catalytic reaction, the product is then passed on to another enzyme.
Control of enzyme activity
The end product(s) of a metabolic pathway are often inhibitors for one of the first enzymes of the pathway (usually the first irreversible step, called committed step), thus regulating the amount of end product made by the pathways. Such a regulatory mechanism is called a negative feedback mechanism, because the amount of the end product produced is regulated by its own concentration. Negative feedback mechanism can effectively adjust the rate of synthesis of intermediate metabolites according to the demands of the cells. This helps with effective allocations of materials and energy economy, and it prevents the excess manufacture of end products. Like other homeostatic devices, the control of enzymatic action helps to maintain a stable internal environment in living organisms.
Errors in metabolism
Since the tight control of enzyme activity is essential for homeostasis, any malfunction (mutation, overproduction, underproduction or deletion) of a single critical enzyme can lead to disease. For example, the most common type of phenylketonuria is caused by a single amino acid mutation in the enzyme phenylalanine hydroxylase, which catalyzes the first step in the degradation of phenylalanine. The resulting build-up of phenylalanine and related products can lead to mental retardation if the disease is untreated [8].
Enzyme-naming conventions
By common convention, an enzyme's name consists of a description of what it does, with the word ending in -ase. Examples are alcohol dehydrogenase and DNA polymerase. Kinases are enzymes that transfer phosphate groups. This results in different enzymes with the same function having the same basic name; they are therefore distinguished by other characteristics, such as their optimal pH (alkaline phosphatase) or their location (membrane ATPase). Furthermore, the reversibility of chemical reactions means that the normal physiological direction of an enzyme's function may not be that observed under laboratory conditions. This can result in the same enzyme being identified with two different names: one stemming from the formal laboratory identification as described above, the other representing its behavior in the cell. For instance the enzyme formally known as xylitol:NAD+ 2-oxidoreductase (D-xylulose-forming) is more commonly referred to in the cellular physiological sense as D-xylulose reductase, reflecting the fact that the function of the enzyme in the cell is actually the reverse of what is often seen under in vitro conditions.
The International Union of Biochemistry and Molecular Biology has developed a nomenclature for enzymes, the EC numbers; each enzyme is described by a sequence of four numbers, preceded by "EC". The first number broadly classifies the enzyme based on its mechanism:
The top-level classification is
- EC 1 Oxidoreductases: catalyze oxidation/reduction reactions
- EC 2 Transferases: transfer a functional group (e.g. a methyl or phosphate group)
- EC 3 Hydrolases: catalyze the hydrolysis of various bonds
- EC 4 Lyases: cleave various bonds by means other than hydrolysis and oxidation
- EC 5 Isomerases: catalyze isomerization changes within a single molecule
- EC 6 Ligases: join two molecules with covalent bonds
The complete nomenclature can be browsed at http://www.chem.qmul.ac.uk/iubmb/enzyme/
Industrial Applications
Application |
Enzymes used |
Uses |
Notes and examples |
Biological detergent | Primarily proteases, produced in an extracellular form from bacteria | Used for presoak conditions and direct liquid applications helping with removal of protein stains from clothes. | |
Amylase enzymes | Detergents for machine dish washing to remove resistant starch residues. | ||
Lipase enzymes | Used to assist in the removal of fatty and oily stains | ||
Cellulase enzymes | Used in biological fabric conditioners | ||
Baking industry | Fungal alpha-amylase enzymes: normally inactivates about 50 degrees Celsius, destroyed during baking process | Catalyze breakdown of starch in the flour to sugar. Yeast action on sugar produces carbon dioxide. Used in production of white bread, buns, and rolls | ![]() |
Protease enzymes | Biscuit manufacturers use them to lower the protein level of flour. | ||
Baby foods | Trypsin | To predigest baby foods | |
Brewing industry | Enzymes from barley are released during the mashing stage of beer production. | They degrade starch and proteins to produce simple sugar, amino acids and peptides that are used by yeast to enhance fermentation. | ![]() |
Industrially produced barley enzymes. | Widely used in the brewing process to substitute for the natural enzymes found in barley. | ||
Amylase, glucanases, proteases | Split polysaccharides and proteins in the malt | ||
Betaglucosidase | Improve the filtration characteristics. | ||
Amyloglucosidase | Low-calorie beer | ||
Proteases | Remove cloudiness during storage of beers. | ||
Fruit juices | Cellulases, pectinases | Clarify fruit juices | |
Dairy industry | Rennin, derived from the stomachs of young ruminant animals (calves, lambs) | Manufacture of cheese, used to split protein | Note: As animals age rennin production decreases and is replaced by another protease, pepsin, which is not suitable for cheese production. In recent years the increase in cheese consumption, as well as increased beef production, has resulted in a shortage of rennin and escalating prices. |
Microbially produced enzyme | Now finding increasing use in the dairy industry | ![]() | |
Lipases | Is implemented during the production of Roquefort cheese to enhance the ripening of the blue-mould cheese. | ||
Lactases | Break down lactose to glucose and galactose | ||
Starch industry | Amylases, amyloglucosideases and glucoamylases | Converts starch into glucose and various syrups | |
Glucose isomerase | Converts glucose into fructose (high fructose syrups derived from starchy materials have enhanced sweetening properties and lower calorific values) | ||
Rubber industry | Catalase | To generate oxygen from peroxide to convert latex to foam rubber | |
Paper industry | Amylases | Degrade starch to lower viscosity product needed for sizing and coating paper | |
Photographic industry | Protease (ficin) | Dissolve gelatin off the scrap film allowing recovery of silver present |
External links

- History of Enzymes, A history of early biotechnological uses of enzymes.
- ExPASy enzyme database, links to Swiss-Prot sequence data, entries in other databases and to related literature searches.
- PDBsum links to the known 3-D structure data of enzymes in the Protein Data Bank.
- MACiE, database of enzyme reaction mechanisms.
- Enzyme Companies
- BRENDA, comprehensive compilation of information and literature references about all known enzymes; requires payment by commercial users.
- Weizmann Institute's Genecards Database, extensive database of protein properties and their associated genes.
- Cytochrome P450 enzymes site lists over 4000 versions of enzymes from this cytochrome in plants and animals.
Further reading
Etymology and history
New Beer in an Old Bottle: Eduard Buchner and the Growth of Biochemical Knowledge, edited by Athel Cornish-Bowden and published by Universitat de València (1997): ISBN 84-370-3328-4, A history of early enzymology.
Williams, Henry Smith, 1863-1943. A History of Science: in Five Volumes. Volume IV: Modern Development of the Chemical and Biological Sciences, A textbook from the 19th century.
Kleyn, J. and Hough J. The Microbiology of Brewing. Annual Review of Microbiology Vol. 25: 583-608
Enzyme structure and mechanism
Structure/Function of Enzymes, Web tutorial on enzyme structure.
Fersht, A. Structure and Mechanism in Protein Science : A Guide to Enzyme Catalysis and Protein Folding. W. H. Freeman, 1998 ISBN 0716732688
Walsh, C., 1979. Enzymatic Reaction Mechanisms. W. H. Freeman and Company.
Page, M. I., and Williams, A. (Eds.), 1987. Enzyme Mechanisms. Royal Society of Chemistry.
Thermodynamics
Reactions and Enzymes Chapter 10 of On-Line Biology Book at Estrella Mountain Community College.
Kinetics and Inhibition
Function and control of enzymes in the cell
Nutritional and Metabolic Diseases
Enzyme-naming conventions
Industrial Applications
References
- ^ Williams, Henry Smith, 1863-1943. A History of Science: in Five Volumes. Volume IV: Modern Development of the Chemical and Biological Sciences
- ^ Dubos, 1<. J. (1951) Louis Pasteur: Free Lance of Science, Gollancz. Quoted in Manchester KL. Louis Pasteur (1822-1895)--chance and the prepared mind. Trends Biotechnol. 1995 Dec;13(12):511-5.
- ^ Biography of Eduard Buchner
- ^ Text of Eduard Buchner's 1907 Nobel lecture
- ^ 1946 Nobel prize for Chemistry laureates
- ^ Mireia Garcia-Viloca,1 Jiali Gao,1 Martin Karplus,2* Donald G. Truhlar Science 9 January 2004: Vol. 303. no. 5655, pp. 186 - 195
- ^ Olsson MH, Siegbahn PE, Warshel A. J Am Chem Soc. 2004 Mar 10;126(9):2820-8.
- ^ Phenylketonuria: NCBI Genes and Disease
- Koshland D. The Enzymes, v. I, ch. 7, Acad. Press, New York, 1959
- Perutz M. Proc. Roy. Soc., B (1967) 167, 448,
- Cha, Y., Murray, C. J. & Klinman, J. P. Science (1989) 243, 1325-1330 .
- Leonor Michaelis and Maud Menten, Die Kinetik der Invertinwirkung, Biochem. Z. (1913) 49, 333-369.
- G. E. Briggs and J. B. S. Haldane, A note on the kinetics of enzyme action, Biochem. J., (1925) 19, 339-339.
- M.V. Volkenshtein, R.R. Dogonadze, A.K. Madumarov, Z.D. Urushadze, Yu.I. Kharkats. Theory of Enzyme Catalysis.- Molekuliarnaya Biologia, (1972), 431-439 (In Russian, English summary)