Formation and evolution of the Solar System
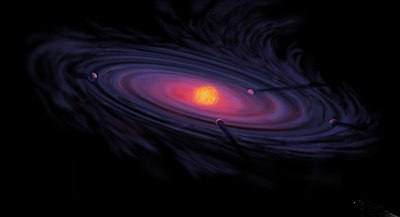
The most widely accepted theory describing the formation and evolution of the Solar System, known as the nebular hypothesis, was first developed in the 18th century by Emanuel Swedenborg, Immanuel Kant, and Pierre-Simon Laplace. The nebular hypothesis maintains that 4.6 billion years ago, the Sun and its planetary system formed from the gravitational collapse of a small part of a giant molecular cloud (a kind of nebula). Since the dawn of the space age and the discovery of extrasolar planets in the 1990s, scientific understanding of planetary formation has been both challenged and refined.
During the collapse of the nebula that formed the Solar System, most of the collapsing mass collected in the centre, forming the Sun; the rest flattened into a protoplanetary disc, out of which the planets and other bodies in the system formed. Similarly, many moons formed from circling discs of gas and dust around their parent planets, but many other moons are believed to have been captured or, in the case of the Earth's Moon, to have resulted from a giant collision. While the Solar System's orbits were once thought to be static, it is now understood that the planets' positions often shift outward or inward over the course of the system's evolution and even switch places. Much of the Solar System's early evolution, in particular the Late Heavy Bombardment, is now believed to be a conseqence of planetary migration.
Just as the Sun and planets were born, they will eventually die. As the Sun begins to age, it will cool and bloat outward to many times its current diameter, becoming a red giant, before casting off its outer layers as a planetary nebula, leaving behind a stellar corpse known as a white dwarf. The planets will follow the Sun's course; in the far distant future, the gravity of passing stars will gradually whittle away at the Sun's retinue of planets. Some will be destroyed, others will be ejected into interstellar space, but ultimately, over the course of trillions of years, the Sun will be left alone and the Solar System will end.
History

Ideas concerning the origin and fate of the world date from the earliest known writings; however, for almost all of that time, there was no attempt to link such theories to the existence of a "Solar System", simply because almost no one knew or believed that the Solar System, in the sense we now understand it, existed. The first step towards a theory of Solar System formation and evolution was the general acceptance of heliocentrism, the model which placed the Sun at the centre of the system and the Earth in orbit around it. This conception had been gestating for millennia, but was only widely accepted by the end of the 17th century. The first recorded use of the term "Solar System" dates from 1704.[1]
The current standard theory for Solar System formation, the nebular hypothesis, has fallen into and out of favour since its formulation by Emanuel Swedenborg, Immanuel Kant and Pierre-Simon Laplace in the 18th century. The usual criticism of the hypothesis has been its apparent inability to explain the Sun's relative lack of angular momentum when compared to the planets.[2] However, studies since the early 1980s of young stars have shown them to be surrounded by cool discs of dust and gas, exactly as the nebular hypothesis predicts, which has led to its re-acceptance.[3]
Understanding of how the Sun will evolve required an understanding of the source of its power. Arthur Stanley Eddington's confirmation of Albert Einstein's theory of relativity led to his realisation that the Sun's energy was driven by nuclear fusion reactions in its core.[4] In 1935, Eddington went further and suggested that other elements might also form within stars.[5] Sir Fred Hoyle was able to elaborate on this premise by showing that all the elements in the universe heavier than helium were in fact created in the hearts of dying stars called red giants. When a red giant finally casts off its outer layers, these elements would then be recycled to form other star systems.[6]
Formation
Pre-solar nebula

The nebular hypothesis maintains that the Solar System formed from the gravitational collapse of fragment of a giant molecular cloud. This initial cloud was likely several light-years across and played host to the birth of several stars.[7] The conventional view is that the Sun formed in relative isolation, but studies of ancient meteorites reveal traces of elements only formed in exploding stars. This indicates that the Sun was born in a region in which a number of supernovae occurred. A shock wave from one of these supernovae may have triggered the formation of the Sun by creating regions of overdensity within the cloud, causing them in turn to collapse[8] and may have altered the composition of the early Solar System by injecting Iron-60.[9]
One of these regions of collapsing gas (known as the pre-solar nebula)[10] would form what became the Solar System. This region had a diameter of between 7000 and 20,000 astronomical units (AU)[7][11][12] and a mass just over that of the Sun. Its composition was about the same as that of the Sun today. Hydrogen and helium, which were produced by Big Bang nucleosynthesis, formed about 98% of the mass. The remaining 2% of the mass consisted of heavier elements that were created by nucleosynthesis in earlier generations of stars.[13] As these stars evolved, they ejected heavier elements into the interstellar medium.[10]
As the nebula collapsed, conservation of angular momentum meant that it spun faster. As the material within the nebula condensed, the atoms within it began to collide with increasing frequency, converting their kinetic energy into heat. The centre, where most of the mass collected, became increasingly hotter than the surrounding disc.[7] As the competing forces associated with gravity, gas pressure, magnetic fields, and rotation acted on it, the contracting nebula began to flatten into a spinning protoplanetary disc with a diameter of roughly 200 AU[7] and a hot, dense protostar (a star in which hydrogen fusion has not yet begun) at the centre.[14]
At this point in its evolution, the Sun is believed to have been a T Tauri star. Studies of T Tauri stars show that they are often accompanied by discs of pre-planetary matter with masses of 0.001–0.1 solar masses.[15] These discs extend to several hundred AU—the Hubble Space Telescope has observed protoplanetary discs up to 1000 AU in star-forming regions such as the Orion Nebula[16]—and are rather cool, reaching only a thousand Kelvin at their hottest.[17] After 100 million years, the temperature and pressure at the core of the Sun became so great that its hydrogen began to fuse, creating an internal source of energy which countered the force of gravitational contraction until hydrostatic equilibrium was achieved. At this point the Sun became a main sequence star.[18]
Formation of planets
The various planets are thought to have formed from the solar nebula, the disc-shaped cloud of gas and dust left over from the Sun's formation.[19] The currently accepted method by which the planets formed is known as accretion, in which the planets began as dust grains in orbit around the central protostar. Through direct contact, these grains formed into clumps between one and ten kilometres (km) in diameter, which in turn collided to form larger bodies (planetesimals) of roughly 5 km in size. These gradually increased through further collisions, growing roughly at the rate of centimetres per year over the course of the next few million years.[20]
The inner Solar System was too warm for volatile molecules like water and methane to condense, so the planetesimals which formed there could only form from compounds with high melting points, such as metals (like iron, nickel, and aluminum) and rocky silicates. These rocky bodies would eventually become the terrestrial planets (Mercury, Venus, Earth, and Mars). These compounds comprised only 0.6% of the mass of the nebula, so the terrestrial planets could not grow very large.[7] The terrestrial embryos grew to about 0.1 Earth masses and ceased accumulating matter about 100,000 years after the formation of the Sun; collisions and mergers allowed terrestrial planets to grow to their present sizes.[21]
The Jovian planets (Jupiter, Saturn, Uranus, and Neptune) formed further out, beyond the the frost line (the point where the Sun's rays are weak enough for volatile icy compounds to remain solid, located between the orbits of Mars and Jupiter). The ices which formed the Jovian planets were more abundant, allowing the planets to grow massive enough to capture hydrogen and helium, the lightest, most abundant elements.[7] Planetesimals beyond the frost line accumulated up to four Earth masses within about 3 million years. Theorists believe it is no accident that Jupiter lies just beyond the frost line. Because the frost line accumulated large amounts of water via evaporation from infalling icy material, it created a region of lower pressure that increased the speed of orbiting dust particles and halted their motion toward the Sun. In effect, the frost line acted as a barrier that caused material to accumulate rapidly at roughly 5 AU from the Sun. This excess material coalesced into a giant planetesimal of about 10 Earth masses which then began to grow rapidly by swallowing hydrogen from the surrounding disc, reaching 150 Earth masses in only another 1000 years and finally topping out at 318 Earth masses. Saturn may owe its substantially lower mass simply to having formed a few million years after Jupiter, when there was less gas available to consume.[21]
Uranus and Neptune are believed to have formed after Jupiter and Saturn did, and likely could never have achieved their current sizes had Jupiter not formed first. After Jupiter attained a certain mass, its gravity created a slingshot effect, accelerating the material ahead and behind it and causing it to move away from the planet. This motion created a gap in the protoplanetary disc. Much like the frost line, this increased speed acted as a barrier that stalled infalling material at a certain distance from the Sun, allowing it to accumulate into larger bodies.[21] Motion in the planetesimal era was not all inward toward the Sun; the Stardust sample return from Comet Wild 2 has suggested that materials from the early formation of the Solar System migrated from the warmer inner Solar System to the region of the Kuiper belt.[22]
Timing
The timeframe of these events has been determined using radiometric dating. Scientists estimate that the Solar System is 4.6 billion years old. The oldest rocks on Earth are approximately 3.9 billion years old. Rocks this old are rare, as Earth's surface is constantly being reshaped by erosion, volcanism and plate tectonics. To estimate the age of the Solar System, scientists use meteorites, which were formed during the early condensation of the solar nebula. The oldest meteorites (such as the Canyon Diablo meteorite) are found to have an age of 4.6 billion years, suggesting that the Solar System must be at least this old.[23]
Studies of discs around other stars have also done much to establish a timeline for Solar System formation. Stars between one and three million years old possess discs rich in gas, whereas discs around stars more than 10 million years old have little to no gas, suggesting that planets within them have ceased forming.[21] T-Tauri stars have far stronger stellar winds than more stable, older stars. By this time, the young Sun's solar wind would have cleared away all the gas and dust in the protoplanetary disc, blowing it into interstellar space, thus ending the growth of the planets.[24][25]
Subsequent evolution
The planets were originally believed to have formed in or near the orbits at which we see them now. However, this view has been undergoing radical change during the late 20th century and the beginning of the 21st century. Currently, it is believed that the Solar System looked very different after its initial formation: several objects at least as massive as Mercury were present in the inner Solar System, the outer Solar System was much more compact than it is now, and the Kuiper belt was much closer to the Sun.[26]
Terrestrial planets
At the end of the planetary formation epoch, the inner Solar System was populated by a large number of Mercury-sized objects. Further growth was possible only because these larger bodies collided and merged, a process which took up to 100 million years. The total radius of the inner Solar System is less than 4 AU, and given that the terrestrial planets are believed to have formed within this region, they could not have migrated significantly over the age of the Solar System.[21] These objects would have gravitationally interacted with one another, tugging at each other's orbits until they collided, growing larger until the four terrestrial planets we know today took shape.[21] One such massive collision is believed to have formed the Moon (see below). How this turbulent period came to an end, leaving the planets with their current near-circular orbits, is still uncertain. One hypothesis is that the growing terrestrials sent a large number of their rivals into the Sun, and with them their random motion. This would have eventually stabilised the terrestrial planets' orbits.[21]
Asteroid belt
Under the solar nebula hypothesis, the asteroid belt initially contained more than enough matter to form 2–3 Earth-like planets, and, indeed, a large number of planetesimals formed there. Later they coalesced and formed 10–20 Moon to Mars-sized planetary embryos;[27] however, Jupiter formed before these embryos could merge into planets.[28] The orbital resonances with Jupiter and Saturn are particularly strong in the asteroid belt, and gravitational interactions with more massive embryos scattered many planetesimals into those resonances. The cumulative action of the resonances and the embryos either scattered the planetesimals away from the asteroid belt or excited their orbital inclinations and eccentricities.[27][29] Eventually those massive embryos too were ejected by Jupiter.[27][30] The effects of giant planets and planetary embryos left the asteroid belt with a total mass equivalent less than 1% that of the Earth, which mainly resided in small planetesimals.[29] This is still 10–20 times more than the current mass in the main belt, which is about 1/2,000 of the Earth's mass.[31]
Planetary migration

The outer two planets are apparently in the "wrong place". Uranus and Neptune (known as the "ice giants") exist in a region where their formation is highly implausible due to the reduced density of the solar nebula and the longer orbital times in their region. They are believed to have originally formed in orbits near Jupiter and Saturn, where more material was available, but to have migrated outward to their current positions over the age of the Solar System. Simulations indicate that gravitational interactions between the less massive outer planets and Jupiter or Saturn can cause the ice giants to scatter either inward or outward, but are more likely to scatter Uranus and Neptune away from the Sun. At larger distances from the Sun, friction caused by the protoplanetary disc tends to make the planets' orbits circular again. Because planetary migration requires that the protoplanetary disc still be present, it must occur while the planets are still forming, within a few million years of the initial formation of the Sun.[33][34]
The Kuiper belt is an outer region of icy bodies which lacked enough mass density to consolidate into a planet, since accretion in its region was too slow to enable planetary formation before the gas dispersed. Today, it is sparsely populated and ranges from 30–55 AU from the Sun, with a farther scattered disc extending to over 100 AU. Originally, however, the Kuiper belt was much denser and closer to the Sun, with an outer edge at approximately 30 AU. Its inner edge would have been just beyond the orbits of Uranus and Neptune, which were in turn far closer to the Sun when they formed (most likely in the range of 15–20 AU), and in opposite locations, with Uranus farther from the Sun than Neptune.[35] Neptune and Uranus interacted with the early Kuiper belt, scattering these small icy bodies inwards, while themselves moving outwards. These planetesimals then scattered off the next planet they encountered in a similar manner, and the next, moving the planets' orbits outwards while the planetesimals moved inwards.[36]
Eventually, Jupiter and Saturn fell into a 2:1 resonance; Saturn orbited the Sun once for every two Jupiter orbits. This resonance created a gravitational push against the outer planets, causing Neptune surge past Uranus and plough into the Kuiper belt, scattering most of the objects. Many of these objects were scattered inwards, until they interacted with Jupiter and most often were placed into highly elliptical orbits or even ejected outright from the Solar System. The objects which ended up in highly elliptical orbits formed the Oort cloud.[37] Closer in, some objects were scattered outwards by Neptune, and those form the scattered disc, accounting for the Kuiper belt's present low mass. However, a large number of Kuiper belt objects, including Pluto, became gravitationally tied to Neptune's orbit, forcing them into resonant orbits.[38]
Late Heavy Bombardment
The migration of Uranus and Neptune and, to lesser extent, of Jupiter and Saturn is thought to be necessary to explain the some features of the Solar System such as the Kuiper belt and Scattered disk. The asteroid belt as well could have been affected by the migrations of the giant planets; disruptions of the main belt could have sent massive numbers of asteroids into the inner Solar System. This could partially account for the belt's current low mass.[29] In addition the disruption of the asteroid belt could explain the late heavy bombardment which occurred approximately 4 billion years ago; 500–600 million years after the formation of the Solar System.[29][39] This period of heavy bombardment lasted several hundred million years and is evident in the cratering still visible on geologically dead bodies of the Solar System such as the Moon and Mercury.[40]
The Late Heavy Bombardment would have devastated the Earth, boiling away any nascent oceans which may have existed on its surface. However, comet collisions in the era following the Bombardment were 1000 times more numerous than today, and could have played a role in bringing the Earth its water.[41][42] Water is too volatile to have formed with the Earth and must have been subsequently delivered from the outer Solar System via comet impacts. Water is essential for the development of life, and the oldest known evidence for life on Earth dates to 3.8 billion years ago—almost immediately after the end of the Late Heavy Bombardment.[43] Earth could only have received 6% of its water from the outer Solar System, however.[44] Work since 2006 by David Jewitt and Henry Hsieh has suggested that the vast majority of the water in Earth's oceans probably derived from a population of comets in the asteroid belt.[45] Although not widely accepted, the panspermia hypothesis holds that life itself may have been deposited on Earth in this way.[46]
Toward the present
Impacts are currently believed to be a regular (if infrequent) part of the evolution of the Solar System. That collisions continue to happen is evidenced by the collision of Comet Shoemaker-Levy 9 with Jupiter in 1994, and the impact feature Meteor Crater in Arizona. The process of accretion, therefore, is not complete, and may still pose a threat to life on Earth.[47][48]
The evolution of the outer Solar System appears to have been influenced by nearby supernovae and possibly also passage through interstellar clouds. The surfaces of bodies in the outer Solar System would experience space weathering from the solar wind, micrometeorites and the neutral components of the interstellar medium.[49]
The evolution of the asteroid belt after Late Heavy Bombardment was mainly governed by collisions.[50] The heavy mass loss is the chief factor that has prevented it from consolidating into a planet. Objects with very large mass have a strong enough gravitational field to prevent the loss of large amounts of material as a result of a violent collision. In the asteroid belt this usually is not the case. As a result, many larger objects have been broken apart, and sometimes newer objects have been forced out of the remnants in less violent collisions.[50] Evidence of collisions can be found in the moons around some asteroids, which currently can only be explained as being consolidations of material flung away from the parent object without enough energy to escape it.[51]
Moons
Moons have come to exist around most planets and many other Solar System bodies. These natural satellites have come into being from one of three possible causes:
- co-formation from a circum-planetary disc (peculiar to the gas giants),
- formation from impact debris (given a large enough impact at a shallow angle), and
- capture of a passing object.
Jupiter and Saturn have a number of large moons, such as Io, Europa, Ganymede and Titan, which may have originated from discs around each giant planet in much the same way that the planets formed around the disc around the Sun.[52] This origin is indicated by the large sizes of the moons and their proximity to the planet. These attributes are impossible to achieve via capture, while the gaseous nature of the primaries make formation from collision debris another impossibility. The outer moons of the gas giants tend to be small and have eccentric orbits with arbitrary inclinations. These features are appropriate for captured bodies.[53][54] Most such moons orbit in the reverse direction to their parent bodies' rotation. The largest irregular moon is Neptune's moon Triton, which is believed to be a captured Kuiper belt object.[55]
Moons of solid Solar System bodies have been created by both collisions and capture. Mars's two small moons, Deimos and Phobos, are believed to be captured asteroids.[56] The Earth's Moon is believed to have formed as a result of a single, large collision.[57] The impacting object likely had a mass comparable to that of Mars, and the impact probably occurred while the Earth was still accreting matter.[58] This Mars-sized object probably formed at one of the stable Earth-Sun Lagrangian points (either L4 or L5) and drifted from its position.[59] A percentage of the material kicked up by the collision ending up in orbit and coalescing into one or more moons.[60] Pluto's moon Charon may also have formed by means of a large collision; the Pluto-Charon and Earth-Moon systems are the only two in the Solar System in which the satellite's mass is at least ~1% that of the larger body.[60]
Future

Astronomers estimate that the Solar System as we know it today will not change drastically until the Sun has fused all the hydrogen fuel in its core into helium, beginning its journey off of the main sequence and into its red giant phase. Even so, the Solar System will continue to evolve as time goes on.
Long-term stability
The Solar System is chaotic,[61] with the orbits of the planets open to long-term variations. One notable example of this chaos is the Neptune-Pluto system, which lies in a 3:2 orbital resonance. Although the resonance itself will remain stable, it becomes impossible to predict the position of Pluto with any degree of accuracy more than 10–20 million years (the Lyapunov time) into the future.[62] The planets' orbits are chaotic over longer timescales, such that the whole Solar System possesses a Lyapunov time in the range of 2–230 million years.[63] In all cases this means that the position of a planet along its orbit ultimately becomes impossible to predict with any certainty (so, for example, the timing of winter and summer become uncertain); but in some cases the orbits themselves may change dramatically. Such chaos manifests most strongly as changes in eccentricity, with some planets' orbits becoming significantly more—or less—elliptical.[64]
Ultimately, the Solar System is stable in that none of the planets will collide with each other or be ejected from the system in the next few billion years.[63] Beyond this, within five billion years or so Mars' eccentricity may grow to around 0.2, such that it lies on an Earth-crossing orbit, leading to a potential collision. In the same timescale, Mercury's eccentricity may grow even further, and a close encounter with Venus could theoretically eject it from the Solar System altogether,[61] or send it on a collision course with Venus or Earth.[65]
Moon-ring systems
The evolution of moon systems is driven by tidal forces. A moon will raise a tidal bulge in the object it orbits (the primary) due to the differential gravitational force across diameter of the primary. If a moon is revolving in the same direction as the planet's rotation and the planet is rotating faster than the orbital period of the moon, the bulge will constantly be pulled ahead of the moon. In response, the moon will gain energy and slowly spiral outward. The same situation will also cause the primary to rotate more slowly over time. The Earth and its moon are just one example of this configuration. Other examples are the Galilean moons of Jupiter (as well as many of Jupiter's smaller moons),[66] and most of the larger moons of Saturn.[67]
Another situation is when the moon is either revolving around the primary faster than the primary rotates, or is revolving in the opposite direction of the planet's rotation. In these cases, the tidal bulge ends up being behind the moon in its orbit. This tidal deceleration causes the moon to spiral in towards the primary until it either is torn apart by tidal stresses, potentially creating a planetary ring system, or crashes into the planet's surface or atmosphere. Such a fate awaits the moons Phobos of Mars, Metis and Adrastea of Jupiter, and Triton of Neptune.[68][69]
A third possibility is where the primary and moon are tidally locked to each other. In that case, the tidal bulge stays directly under the moon and the orbital period will not change. Pluto and Charon are an example of this type of configuration.[70]
Prior to the 2004 arrival of the Cassini-Huygens spacecraft, Saturn's rings were widely thought to be much younger than the Solar System and not expected to survive beyond 300 million years. The gravity from Saturn's moons would gradually sweep the rings' outer edge toward the planet, and, eventually, abrasion by meteorites and Saturn's gravity would take the rest, leaving Saturn unadorned.[71] However, data from the Cassini mission led scientists to revise that early view. Observations revealed 10 km-wide icy clumps of material that repeatedly break apart and reform, keeping the material fresh. Saturn's rings are far more massive than the rings of the other gas giants. This excess mass is believed to have preserved Saturn's rings since Saturn first formed 4.5 billion years ago and is likely to preserve them for billions of years to come.[72]
Evolution of the Sun and planetary environments

In the long-term, the greatest changes in the Solar System will come from changes in the Sun itself as it ages. As the Sun burns through its supply of hydrogen fuel, it gets hotter, and so burns the remaining fuel even faster. As a result, the Sun is growing brighter at a rate of roughly ten percent every 1.1 billion years.[73] In one billion years' time, as the Sun's radiation output increases, its circumstellar habitable zone will move outwards, and the Earth's surface will be seared by solar radiation until it becomes uninhabitable. At this point, all life on land will become extinct.[74] Though life could still survive in the deeper oceans, the salinity of the ocean may sharply increase, potentially ending all life on Earth less than 1 billion years from now.[75] During this time it is possible that as Mars's surface temperature gradually rises, carbon dioxide and water currently frozen under the surface soil will be liberated into the atmosphere, creating a greenhouse effect which will eventually heat up the planet until it achieves parallel conditions to those on Earth today, providing a potential future abode for life.[76] Over the course of a further billion years, Earth's oceans will gradually evaporate, and all life (in known forms) will be impossible. Before 3.5 billion years from now, Earth will attain the surface condition similar to that of Venus today.[73]
Around 4.6 billion years from now, all of the hydrogen in the core of the Sun will have fused into helium. The core will no longer be supported against gravitational collapse and will begin to contract, heating a shell around the core until hydrogen begins to fuse within it.[74] This causes the outer layers of the star to expand greatly, and the star will enter a phase of its life in which it is called a red giant.[77][78] Within 7.5 billion years, the Sun will have expanded to a radius of 1.2 AU—256 times its current size. At the tip of the red giant branch, as a result of the vastly increased surface area, the Sun's surface will be much cooler (about 2600 K) than now and its luminosity much higher (up to 2700 current solar luminosities). For part of its red giant life, the Sun will have a strong stellar wind which will carry away roughly 33% of its mass. The Sun is expected to remain in a red giant phase for about a billion years.[74][79][80]
As the Sun expands, it will most likely swallow up the planets Mercury and Venus. Earth's fate is less clear; although the Sun will eventually envelop Earth's current orbit, the star's loss of mass (and thus weaker gravity) will cause the planets' orbits to move farther out.[74] If it were only for this, Earth would probably escape incineration,[79] but new evidence suggests that Earth will likely be swallowed up as a result of tidal interactions with the Sun's weakly bound outer envelope.[74] During these times, it is possible that the Saturnian moon Titan could achieve surface temperatures akin to those currently required to support life.[81][82]
Gradually, the hydrogen burning in the shell will increase the mass of the core until it reaches about 45% of the present solar mass. At this point the density and temperature will become so high that the fusion of helium into carbon and oxygen will begin, leading to a helium flash; the Sun will shrink abruptly to 11 times its main sequence radius.[74] Simultaneously, its luminosity will decrease to about 53 present its current level, and its temperature will increase to about 4770 K.[74] The Sun will become a horizontal branch (HB) star. The helium-fusing stage will last only 100 million years. Eventually it will have to again resort to its reserves in its outer layers, and will expand again, turning into what is known as an Asymptotic Giant Branch (AGB) star. Here the luminosity of the Sun will increase again, reaching about 2090 present luminosities and its temperature will decrease to about 3500 K.[74] This phase lasts about 30 million years, after which, over the course of a further 100,000 years, the Sun's remaining outer layers will fall away, ejecting a vast stream of matter into space and forming a halo known (misleadingly) as a planetary nebula.[83]
This is a relatively peaceful event; nothing akin to a supernova, which our Sun is too small to undergo as part of its evolution. Any observer present to witness this occurrence would see a massive increase in the speed of the solar wind, but not enough to destroy a planet completely. However, the star's loss of mass could send the orbits of the surviving planets into chaos, causing some to collide, others to be ejected from the Solar System, and still others to be torn apart by tidal interactions.[84] Eventually, all that will remain of the Sun is a white dwarf, an extraordinarily dense object, 54% its original mass but only the size of the Earth. Initially, this white dwarf may be 100 times as luminous as the Sun is now. It will consist only of degenerate carbon and oxygen, but the Sun will never reach temperatures hot enough to fuse these elements, so the white dwarf Sun will gradually cool, growing dimmer and dimmer.[85]
As the Sun dies, its gravitational pull on the orbiting bodies such as planets, comets and asteroids will weaken. All remaining planets' orbits will expand; if Earth still exists by this time, its orbit will lie roughly at 1.85 AU, and Mars' orbit will lie roughly at 2.8 AU. They and the other remaining planets will become dark, frigid hulks, completely devoid of any form of life.[86] They will continue to orbit their star, their speed slowed due to their increased distance from the Sun and the Sun's reduced gravity. Two billion years later, when the Sun has cooled to the 6000–8000K range, the carbon and oxygen in the Sun's core will freeze, with over 90% of its remaining mass assuming a crystalline structure.[87] Eventually, after trillions more years, the Sun will finally cease to shine altogether, becoming a black dwarf.[88]
Galactic evolution
Although the vast majority of galaxies in the Universe are moving away from the Milky Way, the Andromeda Galaxy, the largest member of our Local Group of galaxies, is heading towards us at a speed of roughly 120 km/s.[89] In roughly 2 billion years, Andromeda and the Milky Way will collide, causing both to deform as tidal forces distort their outer arms into vast tidal tails. When this initial disruption occurs, astronomers calculate a 12% chance that the Solar System will be pulled outward into the Milky Way's tidal tail and a 3% chance that it will become gravitationally bound to Andromeda and thus a part of that galaxy.[89] After a further series of glancing blows, during which the likelihood of the Solar System's ejection rises to 30%, the galaxies' supermassive black holes will merge, transforming into an active galactic nucleus, and their tides will cause disruptions in stellar nurseries, leading to a short period of massive star formation called a starburst.[89]
The force of these interactions will likely push the Solar System into the new galaxy's outer halo, leaving it relatively unscathed by the radiation from these collisions. Eventually, in roughly 7 billion years, the Milky Way and Andromeda will complete their merger into a giant elliptical galaxy.[89][90] It is a common misconception that this collision will disrupt the orbits of the planets in the Solar System. While it is true that the gravity of passing stars tugs at planetary orbits, detaching planets from their orbits and sending them into interstellar space, distances between stars are so great that the likelihood of the Milky Way-Andromeda collision causing such disruption to any individual star system is negligible. While the Solar System as a whole could be affected by these events, the Sun and planets are not expected to be disturbed.[91]
However, over time, the probability of a chance encounter with a star increases, and planetary disruption eventually becomes all but inevitable. Assuming that the Big Crunch or Big Rip scenarios for the end of the universe will not occur, probability calculations suggest that the gravity of passing stars will have completely stripped the Sun of planets within 1 quadrillion years. This point marks the end of the Solar System. While the Sun and planets, drifting alone through the galaxy, will survive until all matter collapses into black holes, the Solar System, in any meaningful sense, will cease to exist.[92]
See also
References
- ^ ""Solar"". etymoline. Retrieved 2008-04-15.
- ^ Woolfson, M. M. (1984). "Rotation in the Solar System". Philosophical Transactions of the Royal Society of London. 313: 5.
- ^ Nigel Henbest (1991). "Birth of the planets: The Earth and its fellow planets may be survivors from a time when planets ricocheted around the Sun like ball bearings on a pinball table". New Scientist. Retrieved 2008-04-18.
- ^ David Whitehouse (2005). The Sun: A Biography. John Wiley and Sons.
- ^ Simon Mitton (2005). "Origin of the Chemical Elements". Fred Hoyle: A Life in Science. Aurum. pp. 197–222.
- ^ Simon Mitton (2005). "Origin of the Chemical Elements". Fred Hoyle: A Life in Science. Aurum. pp. 197–222.
- ^ a b c d e f Zabludoff, Ann (University of Arizona) (Spring 2003). "Lecture 13: The Nebular Theory of the origin of the Solar System". Retrieved 2006-12-27.
- ^ Jeff Hester; et al. (21 May 2004). "The Cradle of the Solar System". Science: 1116–1117. doi:10.1126/science.1096808. Retrieved 2007-01-11.
{{cite journal}}
: Check date values in:|date=
(help); Explicit use of et al. in:|author=
(help)CS1 maint: date and year (link) - ^ Bizzarro, Martin (2007). "Evidence for a Late Supernova Injection of 60Fe into the Protoplanetary Disk". Science. 316 (5828): 1178–1181. doi:10.1126/science.1141040.
{{cite journal}}
: Unknown parameter|coauthors=
ignored (|author=
suggested) (help); Unknown parameter|month=
ignored (help) - ^ a b Irvine, W. M. (November 15–19). "The chemical composition of the pre-solar nebula". Cometary exploration; Proceedings of the International Conference, Budapest, Hungary: 3–12. Retrieved 2007-02-15.
{{cite journal}}
: Check date values in:|date=
and|year=
/|date=
mismatch (help); More than one of|work=
and|journal=
specified (help); Unknown parameter|Volume=
ignored (|volume=
suggested) (help) - ^ An astronomical unit, or AU, is the average distance between the Earth and the Sun, or roughly 150 million kilometres. It is the standard unit of measurement for interplanetary distances.
- ^ J. J. Rawal (January, 1986). "Further Considerations on Contracting Solar Nebula" (PDF). Earth, Moon, and Planets. 34 (1). Springer Netherlands: 93–100. Retrieved 2006-12-27.
{{cite journal}}
: Check date values in:|date=
and|year=
/|date=
mismatch (help); More than one of|work=
and|journal=
specified (help); Text "10.1007/BF00054038" ignored (help); Text "doi" ignored (help) - ^ Zeilik & Gregory (1998, p. 207)
- ^ Greaves, Jane S. (2005). "Disks Around Stars and the Growth of Planetary Systems". Science. 307: 68. doi:10.1126/science.1101979.
- ^ Momose, M.; Kitamura, Y.; Yokogawa, S.; Kawabe, R.; Tamura, M.; Ida, S. (2003). "Investigation of the Physical Properties of Protoplanetary Disks around T Tauri Stars by a High-resolution Imaging Survey at lambda = 2 mm" (PDF). In Ikeuchi, S., Hearnshaw, J. and Hanawa, T. (eds.) (ed.). The Proceedings of the IAU 8th Asian-Pacific Regional Meeting, Volume I. Vol. 289. Astronomical Society of the Pacific Conference Series. p. 85.
{{cite conference}}
:|editor=
has generic name (help); Unknown parameter|booktitle=
ignored (|book-title=
suggested) (help)CS1 maint: multiple names: authors list (link) - ^ Deborah L. Padgett, Deborah L.; Brandner, Wolfgang Brandner, Karl R. Stapelfeldt; et al. (1999). "HUBBLE SPACE TELESCOPE/NICMOS Imaging of Disks and Envelopes around Very Young Stars". The Astronomical Journal. 117: 1490–1504. doi:10.1086/300781.
{{cite journal}}
: Explicit use of et al. in:|author=
(help); Unknown parameter|month=
ignored (help)CS1 maint: multiple names: authors list (link) - ^ Küker, M.; Henning, T.; Rüdiger, G. (2003). "Magnetic Star-Disk Coupling in Classical T Tauri Systems". Astrophysical Journal. 589: 397. doi:10.1086/374408.
{{cite journal}}
: CS1 maint: multiple names: authors list (link) - ^ Chrysostomou, A.; Lucas, P. W. (2005). "The Formation of Stars". Contemporary Physics. 46: 29.
{{cite journal}}
: CS1 maint: multiple names: authors list (link) - ^ A. P. Boss and R. H. Durisen (2005). "Chondrule-forming Shock Fronts in the Solar Nebula: A Possible Unified Scenario for Planet and Chondrite Formation". The Astrophysical Journal. 621: L137–L140.
{{cite journal}}
: Text "doi: 10.1086/429160" ignored (help) - ^ Goldreich, P.; Ward, W. R. (1973). "The Formation of Planetesimals". Astrophysical Journal. 183: 1051. Retrieved 2006-11-16.
{{cite journal}}
: CS1 maint: multiple names: authors list (link) - ^ a b c d e f g Douglas N. C. Lin (May 2008). "The Genesis of Planets" (fee required). Scientific American. 298 (5): 50–59.
- ^ Emily Lakdawalla (2006). "Stardust Results in a Nutshell: The Solar Nebula was Like a Blender". The Planetary Society. Retrieved 2007-01-02.
- ^ Wallace Gary Ernst (2000). "Earth's Place in the Solar System". Earth Systems: Processes and Issues. Cambridge University Press. pp. 45–58. Retrieved 2008-04-04.
- ^ Elmegreen, B. G. (1979). "On the disruption of a protoplanetary disc nebula by a T Tauri like solar wind". Astronomy & Astrophysics. 80: 77. Retrieved 2006-11-19.
- ^ Heng Hao (Harvard University) (November 24, 2004). "Disc-Protoplanet interactions" (PDF). Retrieved 2006-11-19.
{{cite web}}
: Check date values in:|date=
(help) - ^ Mike Brown (California Institute of Technology ). "Dysnomia, the moon of Eris". Personal web site. Retrieved 2008-02-01.
- ^ a b c Bottke, William F. (2005). "Linking the collisional history of the main asteroid belt to its dynamical excitation and depletion" (pdf). Icarus. 179: 63–94. doi:10.1016/j.icarus.2005.05.017.
{{cite journal}}
: Unknown parameter|coauthors=
ignored (|author=
suggested) (help) - ^ Petit, Jean-Marc (2001). "The Primordial Excitation and Clearing of the Asteroid Belt" (pdf). Icarus. 153: 338–347. doi:10.1006/icar.2001.6702.
{{cite journal}}
: Unknown parameter|coauthors=
ignored (|author=
suggested) (help) - ^ a b c d O’Brien, David P. (2007). "The primordial excitation and clearing of the asteroid belt—Revisited" (pdf). Icarus. 191: 434–452. doi:10.1016/j.icarus.2007.05.005.
{{cite journal}}
: Unknown parameter|coauthors=
ignored (|author=
suggested) (help) - ^ Watanabe, Susan (July 20, 2001). "Mysteries of the Solar Nebula". NASA. Retrieved 2007-04-02.
{{cite web}}
: Check date values in:|date=
(help) - ^ Krasinsky, G. A. (2002). "Hidden Mass in the Asteroid Belt". Icarus. 158 (1): 98–105. doi:10.1006/icar.2002.6837.
{{cite journal}}
: Unknown parameter|coauthors=
ignored (|author=
suggested) (help); Unknown parameter|month=
ignored (help) - ^ Gomes, Levison, Tsiganis, Morbidelli (2005). "Origin of the cataclysmic Late Heavy Bombardment period of the terrestrial planets" (PDF). Nature. 435: 466. doi:doi:10.1038/nature03676.
{{cite journal}}
: Check|doi=
value (help)CS1 maint: multiple names: authors list (link) - ^ Thommes, E. W. (2002). "The Formation of Uranus and Neptune among Jupiter and Saturn". Astronomical Journal. 123: 2862. doi:10.1086/339975. arXiv:astro-ph/0111290.
{{cite journal}}
: Unknown parameter|coauthors=
ignored (|author=
suggested) (help) - ^ Fogg, M. J. (2007). "On the formation of terrestrial planets in hot-Jupiter systems". Astronomy & Astrophysics. 461: 1195. doi:10.1051/0004-6361:20066171. arXiv:astro-ph/0610314.
{{cite journal}}
: Unknown parameter|coauthors=
ignored (|author=
suggested) (help) - ^ Origin of the cataclysmic Late Heavy Bombardment period of the terrestrial planets, Gomes, Levison, Tsiganis, Morbidelli — Nature 26, May 2005.
- ^ "Uranus, Neptune, and the Mountains of the Moon". Planetary Science Research Discoveries,. 21 August 2001. Retrieved 2008-02-01.
{{cite web}}
: CS1 maint: extra punctuation (link) - ^ "Kuiper belt and Oort cloud". nineplanets.org. Retrieved 2008-03-13.
- ^ Malhotra, R. (1995). "The Origin of Pluto's Orbit: Implications for the Solar System Beyond Neptune". Astronomical Journal. 110: 420. doi:10.1086/117532. arXiv:astro-ph/9504036.
- ^ Kathryn Hansen (2005). "Orbital shuffle for early solar system". Geotimes. Retrieved 2006-06-22.
- ^ "Chronology of Planetary surfaces". NASA History Division. Retrieved 2008-03-13.
- ^ Francis Reddy (2006). "New comet class in Earth's backyard". astronomy.com. Retrieved 2008-04-29.
- ^ Morbidelli, A.; Chambers, J.; Lunine, J. I.; Petit, J. M.; Robert, F.; Valsecchi, G. B.; Cyr, K. E. (2000). "Source regions and timescales for the delivery of water to the Earth". Meteoritics & Planetary Science. 35: 1309. ISSN 1086-9379.
{{cite journal}}
: CS1 maint: multiple names: authors list (link) - ^ "UCLA scientists strengthen case for life more than 3.8 billion years ago". University of California. 2006. Retrieved 2008-04-29.
- ^ R. Gomes, H. F. Levison, K. Tsiganis & A. Morbidelli (26 May 2005). "Origin of the cataclysmic Late Heavy Bombardment period of the terrestrial planets". Nature. 435. doi:10.1038/nature03676. Retrieved 2008-04-04.
{{cite journal}}
: Check date values in:|date=
(help)CS1 maint: multiple names: authors list (link) - ^ Henry H. Hsieh and David Jewitt (23 March 2006). "A Population of Comets in the Main Asteroid Belt". Science. 312 (5773): 561–563. doi:10.1126/science.1125150. Retrieved 2008-04-05.
- ^ Florence Raulin-Cerceau, Marie-Christine Maurel and Jean Schneider (1998). "From Panspermia to Bioastronomy, the Evolution of the Hypothesis of Universal Life". Origins of Life and Evolution of Biospheres. 28. Springer Netherlands: 597–612. doi:10.1023/A:1006566518046. Retrieved 2007-12-19.
- ^ Clark R. Chapman (2 Feb. 1995). "The Risk to Civilization From Extraterrestrial Objects and Implications of the Shoemaker-Levy 9 Comet Crash". Abhandlungen der Geologischen Bundeanstalt, Wien,. 53: 51–54. Retrieved 2008-04-02.
{{cite journal}}
: Check date values in:|date=
and|year=
/|date=
mismatch (help); More than one of|work=
and|journal=
specified (help)CS1 maint: extra punctuation (link) - ^ Craig B. Agnor & Douglas P. Hamilton (2006). "Neptune's capture of its moon Triton in a binary-planet gravitational encounter" (PDF). Nature. Retrieved 2006-06-20.
- ^ Beth E. Clark, Robert E. Johnson (1996). "Interplanetary Weathering: Surface Erosion in Outer Space". Astronomy Department, Cornell University, Ithaca, New York; Engineering Physics, University of Virginia, Charlottesville. Retrieved 2008-03-13.
- ^ a b Bottke, William F. (2005). "The origin and evolution of stony meteorites" (pdf). Proceedings of the International Astronomical Union. Dynamics of Populations of Planetary Systems. Vol. 197. pp. 357–374. doi:10.1017/S1743921304008865.
{{cite conference}}
: Unknown parameter|booktitle=
ignored (|book-title=
suggested) (help); Unknown parameter|coauthors=
ignored (|author=
suggested) (help) - ^ Alfvén, H.; Arrhenius, G. (1976). "The Small Bodies". SP-345 Evolution of the Solar System. NASA. Retrieved 2007-04-12.
{{cite web}}
: CS1 maint: multiple names: authors list (link) - ^ Takato, N.; Bus, S. J.; Terada, H.; Pyo, T.-S.; Kobayashi, N. (2004). "Detection of a Deep 3-m Absorption Feature in the Spectrum of Amalthea (JV)". Science. 306: 2224. doi:10.1126/science.1105427.
{{cite journal}}
: CS1 maint: multiple names: authors list (link)
See also Cain, Fraser (December 24, 2004). "Jovian Moon Was Probably Captured". Universe Today. Retrieved 2008-04-03.{{cite news}}
: Check date values in:|date=
(help) - ^ Jewitt, D.C.; Sheppard, S.; Porco, C. (2004). "Jupiter's outer satellites and Trojans". In Fran Bagenal, Timothy E. Dowling, William B. McKinnon (ed.). Jupiter. The Planet, Satellites and Magnetosphere. Cambridge University Press. pp. 263–280. ISBN 0-521-81808-7.
{{cite conference}}
: Unknown parameter|booktitle=
ignored (|book-title=
suggested) (help)CS1 maint: multiple names: authors list (link) (PDF retrieved on March 28, 2008.) - ^ Scott S. Sheppard (Carnegie Institution of Washington). "The Giant Planet Satellite and Moon Page". Personal web page. Retrieved 2008-03-13.
- ^ Craig B Agnor, Douglas P Hamilton (May 2006). "Neptune's capture of its moon Triton in a binary–planet gravitational encounter". Nature. 441 (7090): 192–194. doi:10.1038/nature04792. Retrieved 2006-05-10.
{{cite journal}}
: CS1 maint: date and year (link) - ^ Zeilik & Gregory (1998, pp. 118–120)
- ^ Stevenson, D. J. (1987). "Origin of the moon — The collision hypothesis". Annual Review of Earth and Planetary Sciences. 15: 271. doi:10.1146/annurev.ea.15.050187.001415.
- ^ Canup, R. M.; Asphaug, E. (2001). "Origin of the Moon in a giant impact near the end of the Earth's formation". Nature. 412: 708. doi:10.1038/35089010.
{{cite journal}}
: CS1 maint: multiple names: authors list (link) - ^ Taylor, G. Jeffrey (December 31, 1998). "Origin of the Earth and Moon". Planetary Science Research Discoveries (PSRD). Hawaii Institute of Geophysics & Planetology. Retrieved 2007-07-25.
{{cite web}}
: Check date values in:|date=
(help) - ^ a b Robin M. Canup (28 January 2005). "A Giant Impact Origin of Pluto-Charon". Science. 307 (5709): 546–550. doi:10.1126/science.1106818. Retrieved 2008-05-01.
{{cite journal}}
: Check date values in:|date=
(help) - ^ a b Laskar, J (1994). "Large-scale chaos in the solar system". Astronomy and Astrophysics. 287: L9–L12.
- ^ Gerald Jay Sussman; Jack Wisdom (1988). "Numerical evidence that the motion of Pluto is chaotic". Science. 241: 433–437.
{{cite journal}}
: CS1 maint: multiple names: authors list (link) - ^ a b Hayes, W. B. (2007). "Is the outer Solar System chaotic?". Nature Physics. 3: 689–691. doi:10.1038/nphys728. arXiv:astro-ph/0702179.
- ^ Stewart, Ian (1997). Does God Play Dice? (2nd ed.). Penguin Books. pp. 246–249. ISBN 0-14-025602-4.
- ^ "The solar system could go haywire before the sun dies". New Scientist. 23 April 2008. Retrieved 2008-04-28.
- ^ A. Gailitis (1980). "Tidal Heating of Io and orbital evolution of the Jovian satellites". Latvian Academy of Sciences. Retrieved 2008-03-27.
- ^ Bevilacqua, R. (1980). "Resonances and close approaches. I. The Titan-Hyperion case". Earth, Moon, and Planets. 22 (2): 141–152. Retrieved 2007-08-27.
{{cite journal}}
: Unknown parameter|coauthors=
ignored (|author=
suggested) (help); Unknown parameter|month=
ignored (help) - ^ Chyba, C. F.; Jankowski, D. G.; Nicholson, P.D. (1989). "Tidal evolution in the Neptune-Triton system". Retrieved 2007-03-03.
{{cite web}}
: CS1 maint: multiple names: authors list (link) - ^
Holsapple, K. A. (2001). "Equilibrium Configurations of Solid Cohesionless Bodies". Icarus. 154 (2): 432–448. doi:10.1006/icar.2001.6683. Retrieved 2007-11-12.
{{cite journal}}
: Unknown parameter|month=
ignored (help) - ^ Marc Buie; William Grundy; Eliot Young; Leslie Young; Alan Stern (2006). "Orbits and Photometry of Pluto's Satellites: Charon, S/2005 P1, and S/2005". Retrieved 2008-03-13.
{{cite web}}
: Text "The Astronomical Journal" ignored (help)CS1 maint: multiple names: authors list (link) - ^ Stefano Coledan (2002). "Saturn Rings Still A Mystery". Popular Mechanics. Retrieved 2007-03-03.
- ^ "Saturn's recycled rings". Astronomy Now (February 2008): 9.
- ^ a b JEFF HECHT (1994). "Science: Fiery future for planet Earth". NewScientist. Retrieved 2007-10-29.
- ^ a b c d e f g h Schroder, K.P. (2008). "Distant future of the Sun and Earth revisited". Mon. Not. R. Astron. Soc. 386: 155–163. doi:10.1111/j.1365-2966.2008.13022.x.
{{cite journal}}
: Unknown parameter|coauthors=
ignored (|author=
suggested) (help)CS1 maint: unflagged free DOI (link) - ^ Knut Jørgen and Røed Ødegaard (2004). "Our changing solar system". Centre for International Climate and Environmental Research. Retrieved 2008-03-27.
- ^ J. S. (Jeffrey Stuart) Kargel. "Mars: A Warmer, Wetter Planet". Retrieved 2007-10-29.
- ^ Zeilik & Gregory (1998, p. 320–321)
- ^ "Introduction to Cataclysmic Variables (CVs)". NASA Goddard Space Center. 2006. Retrieved 2006-12-29.
- ^ a b I.J. Sackmann, A.I. Boothroyd, K.E. Kraemer (1993). "Our Sun. III. Present and Future". Astrophysical Journal. 418: 457.
{{cite journal}}
: CS1 maint: multiple names: authors list (link) - ^ Zeilik & Gregory (1998, p. 322)
- ^ Ralph D. Lorenz, Jonathan I. Lunine, Christopher P. McKay (1997). "Titan under a red giant sun: A new kind of "habitable" moon" (PDF). Geophysical Research Letters. 24: 2905. Retrieved 2008-03-21.
{{cite journal}}
: CS1 maint: multiple names: authors list (link) - ^ Marc Delehanty. "Sun, the solar system's only star". Astronomy Today. Retrieved 2006-06-23.
- ^ Bruce Balick (Department of Astronomy, University of Washington). "Planetary nebulae and the future of the Solar System". Personal web site. Retrieved 2006-06-23.
- ^ B. T. Gänsicke, T. R. Marsh, J. Southworth, A. Rebassa-Mansergas (22 December 2006). "A Gaseous Metal Disk Around a White Dwarf". Science. 314 (5807): 1908–1910. doi:10.1126/science.1135033.
{{cite journal}}
: CS1 maint: multiple names: authors list (link) - ^ Pogge, Richard W. (1997). "The Once & Future Sun" (lecture notes). New Vistas in Astronomy. Retrieved 2005-12-07.
{{cite web}}
: External link in
(help)|work=
- ^ Sackmann, I.-Juliana (1993). "Our Sun. III. Present and Future". Astrophysical Journal. 418: 457.
{{cite journal}}
: Unknown parameter|coauthors=
ignored (|author=
suggested) (help); Unknown parameter|month=
ignored (help) - ^ A Kanaan, A Nitta, DE Winget; et al. (2004). "Testing White Dwarf Crystallization Theory with Asteroseismology of the Massive Pulsating DA Star BPM 37093". The Astrophysical Journal. Retrieved 2008-01-17.
{{cite web}}
: Explicit use of et al. in:|author=
(help)CS1 maint: multiple names: authors list (link) - ^ Richard W. Pogge (1997). "The Once and Future Sun". Perkins Observatory. Retrieved 2006-06-23.
- ^ a b c d Fraser Cain (2007). "When Our Galaxy Smashes Into Andromeda, What Happens to the Sun?". Universe Today. Retrieved 2007-05-16.
- ^ Cox, T. J.; Loeb, Abraham (2007). "The Collision Between The Milky Way And Andromeda". Montly Notices of the Royal Astronomical Society. doi:10.1111/j.1365-2966.2008.13048.x. arXiv:0705.1170. Retrieved 2008-04-02.
{{cite journal}}
: CS1 maint: multiple names: authors list (link) CS1 maint: unflagged free DOI (link) - ^ J. Braine, U. Lisenfeld, P. A. Duc, E. Brinks, V. Charmandaris and S. Leon (2004). "Colliding molecular clouds in head-on galaxy collisions". Astronomy and Astrophysics. 418: 419–428. Retrieved 2008-04-02.
{{cite journal}}
: Text "doi: 10.1051/0004-6361:20035732" ignored (help)CS1 maint: multiple names: authors list (link) - ^ Freeman Dyson (July 1979). "Time Without End: Physics and Biology in an open universe". Reviews of Modern Physics. 51 (3). Retrieved 2008-04-02.
{{cite journal}}
: More than one of|work=
and|journal=
specified (help)
Further reading
- Lissauer, J. J. (2006), "Planet Formation, Protoplanetary Disks and Debris Disks", in L. Armus and W. T. Reach (ed.), The Spitzer Space Telescope: New Views of the Cosmos, vol. 357, Astronomical Society of the Pacific Conference Series, p. 31
- Woolfson, M. M. (1993), "The Solar System: Its Origin and Evolution", Journal of the Royal Astronomical Society, 34: 1–20, retrieved 2008-04-16
{{citation}}
: More than one of|work=
and|journal=
specified (help) - Zeilik, Michael A.; Gregory, Stephan A. (1998), Introductory Astronomy & Astrophysics (4th ed.), Saunders College Publishing, ISBN 0030062284
External links
- 7M animation from skyandtelescope.com showing the early evolution of the outer Solar System.
- Quicktime animation of the future collision between the Milky Way and Andromeda