Mass
![]() | This article has multiple issues. Please help improve it or discuss these issues on the talk page. (Learn how and when to remove these template messages)
No issues specified. Please specify issues, or remove this template. |

In physics, mass (from Greek μάζα) commonly refers to any of three properties of matter, which have been shown experimentally to be equivalent: inertial mass, active gravitational mass and passive gravitational mass. In everyday usage, mass is often taken to mean [matter]].
The inertial mass of an object determines its acceleration in the presence of an applied force. According to Isaac Newton's second law of motion, if a body of mass m is subjected to a force F, its acceleration a is given by F/m.
A body's mass also determines the degree to which it generates or is affected by a gravitational field. If a first body of mass m1 is placed at a distance r from a second body of mass m2, the first body experiences an attractive force F given by
where G is the universal constant of gravitation, equal to 6.67×10−11 kg−1 m3 s−2. This is sometimes referred to as gravitational mass (when a distinction is necessary, M is used to denote the active gravitational mass and m the passive gravitational mass). Repeated experiments since the seventeenth century have demonstrated that inertial and gravitational mass are equivalent; this is entailed in the equivalence principle of general relativity.
On the surface of the Earth, the weight W of an object is related to its mass m by
where g is the acceleration due to the Earth's gravity, equal to 9.81 m s−2. An object's weight depends on its location, while its mass does not: an object with a mass of 50 kilograms weighs 491 newtons on the surface of the Earth; on the surface of the Moon, the same object still has a mass of 50 kilograms but weighs only 81.5 newtons.
An object with mass is said to be massive.[1]
Units of mass
In the International System of Units (SI), mass is measured in kilograms (kg). The gram (g) is 1⁄1000 of a kilogram.
Other units are accepted for use in SI:
- The tonne (t) is equal to 1000 kg.
- The electronvolt (eV) is primarily a unit of energy, but because of the mass-energy equivalence it can also function as a unit of mass. In this context it is denoted eV/c2, or simply as eV. The electronvolt is common in particle physics.
- The atomic mass unit (u) is defined so that a single carbon-12 atom has a mass of 12 u; 1 u is approximately 1.66×10−27 kg.[note 1] The atomic mass unit is convenient for expressing the masses of atoms and molecules.
Outside the SI system, a variety of different mass units are used, depending on context, such as the slug (sl), the pound (lb), the Planck mass (mP), and the solar mass (M⊙).
In normal situations, the weight of an object is proportional to its mass, which usually makes it unproblematic to use the same unit for both concepts. However, the distinction between mass and weight becomes important for measurements with a precision better than a few percent (because of slight differences in the strength of the Earth's gravitational field at different places), and for places far from the surface of the Earth, such as in space or on other planets.
A mass can sometimes be expressed in terms of length. Here one identifies the mass of a particle with its inverse Compton wavelength (1 cm−1 ≈ 3.52×10−41 kg).
Summary of mass concepts and formalisms
In classical mechanics, mass has a central role in determining the behavior of bodies. Newton's second law relates the force F exerted in a body of mass m to the body's acceleration a:
Additionally, mass relates a body's momentum p to its velocity v:
and the body's kinetic energy Ek to its velocity:
In special relativity, relativistic mass is a formalism which accounts for relativistic effects by having the mass increase with velocity.
Since energy is dependent on reference frame (upon the observer) it is convenient to formulate the equations of physics in a way such that mass values are invariant (do not change) between observers, and so the equations are independent of the observer. For a single particle, this quantity is the rest mass; for a system of bound or unbound particles, this quantity is the invariant mass. The invariant mass m of a body is related to its energy E and the magnitude of its momentum p by
- where c is the speed of light.
Summary of mass related phenomena
Template:Properties of mass In physical science, one may distinguish conceptually between at least seven attributes of mass, or seven physical phenomena that can be explained using the concept of mass:[2]
- Inertial mass is a measure of an object's resistance to changing its state of motion when a force is applied. It is determined by applying a force to an object and measuring the acceleration that results from that force. An object with small inertial mass will accelerate more than an object with large inertial mass when acted upon by the same force. One says the body of greater mass has greater inertia.
- The amount of matter in certain types of samples can be exactly determined through electrodeposition or other precise processes. The mass of an exact sample is determined in part by the number and type of atoms or molecules it contains, and in part by the energy involved in binding it together (which contributes a negative "missing mass," or mass deficit).
- Active gravitational mass is a measure of the strength of an object’s gravitational flux (gravitational flux is equal to the surface integral of gravitational field over an enclosing surface). Gravitational field can be measured by allowing a small ‘test object’ to freely fall and measuring its free-fall acceleration. For example, an object in free-fall near the Moon will experience less gravitational field, and hence accelerate slower than the same object would if it were in free-fall near the earth. The gravitational field near the Moon is weaker because the Moon has less active gravitational mass.
- Passive gravitational mass is a measure of the strength of an object's interaction with a gravitational field. Passive gravitational mass is determined by dividing an object’s weight by its free-fall acceleration. Two objects within the same gravitational field will experience the same acceleration; however, the object with a smaller passive gravitational mass will experience a smaller force (less weight) than the object with a larger passive gravitational mass.
- Energy also has mass according to the principle of mass–energy equivalence. This equivalence is exemplified in a large number of physical processes including pair production, nuclear fusion, and the gravitational bending of light. Pair production and nuclear fusion are processes through which measurable amounts of mass and energy are converted into each other. In the gravitational bending of light, photons of pure energy are shown to exhibit a behavior similar to passive gravitational mass.
- Curvature of spacetime is a relativistic manifestation of the existence of mass. Curvature is extremely weak and difficult to measure. For this reason, curvature wasn’t discovered until after it was predicted by Einstein’s theory of general relativity. Extremely precise atomic clocks on the surface of the earth, for example, are found to measure less time (run slower) than similar clocks in space. This difference in elapsed time is a form of curvature called gravitational time dilation. Other forms of curvature have been measured using the Gravity Probe B satellite.
- Quantum mass manifests itself as a difference between an object’s quantum frequency and its wave number. The quantum mass of an electron, the Compton wavelength, can be determined through various forms of spectroscopy and is closely related to the Rydberg constant, the Bohr radius, and the classical electron radius. The quantum mass of larger objects can be directly measured using a watt balance. (Note: Quantum mass can be represented in many different mathematical formalisms. The Schrödinger equation is a classical formalism in which kinetic energy is equal to the classical momentum squared divided by the classical mass. The Klein–Gordon equation is a relativistic formalism in which the invariant mass is equal to the difference of the energy squared and the momentum squared.)
Inertial mass, passive and active gravitational mass, and the various other mass-related phenomena are conceptually distinct. However, every experiment to date has shown these values to be proportional, and this proportionality gives rise to the abstract concept of mass. If, in some future experiment, one of the mass-related phenomena is shown to not be proportional to the others, then that specific phenomena will no longer be considered a part of the abstract concept of mass.
Weight and amount
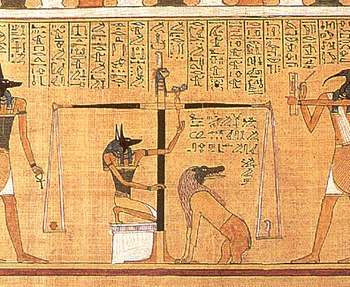
The concepts of passive gravitational mass and atomic mass grew out of the much older concepts of weight and amount. Weight, by definition, is a measure of the force that must be applied to support an object (i.e. hold it at rest) in a gravitational field. Gravitational fields typically change only slightly over short distances, and the gravitational field of the earth is nearly uniform at all locations on the earth’s surface; therefore, an object’s weight changes only slightly when it is moved from one location to another, and these changes went unnoticed through much of history. This may have given early humans the impression that weight was unchanging, and a fundamental property of all objects.
In the Egyptian religious illustration to the right, Anubis is using a balance scale to weigh the heart of Hunefer. A balance scale balances the force of one object’s weight against the force of another object’s weight. When two objects are near each other, they experience almost identical gravitational fields. Hence, if two nearby objects have similar masses then their weights will also be similar. The two sides of a balance scale are close enough that the scale, by comparing weights, also compares passive gravitational masses. Hence, the balance scale measures mass and is one of the oldest known devices used for this purpose.
The concept of amount is very old and predates recorded history, so any description of the early development of this concept is speculative in nature. However, one might reasonably assume that humans, at some early era, realized that the weight of a collection of similar objects was directly proportional to the number of objects in the collection:
- ,
where is the weight of the collection of similar objects and is the number of objects in the collection. Proportionality, by definition, implies that two values have a constant ratio:
- , or equivalently .
Consequently, historical weight standards were often defined in terms of amounts. The Romans, for example, used the carob seed (carat or siliqua) as a measurement standard. If an object’s weight was found to be equivalent to 1728 carob seeds, then the object was said to weigh one Roman pound. If, on the other hand, the object’s weight was found to be equivalent to 144 carob seeds then the object was said to weigh one Roman ounce (uncia). The Roman pound and ounce were both defined in terms of different sized collections of the same common mass standard, the carob seed. The ratio of a Roman ounce (144 carob seeds) to a Roman pound (1728 carob seeds) was:
- .
This example illustrates a fundamental principle of physical science: when values are related through simple fractions, there is a good possibility that the values stem from a common source.
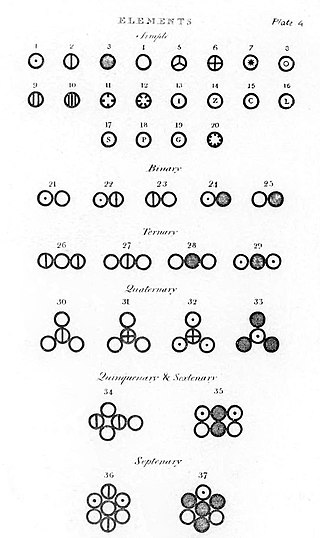
The name atom comes from the Greek ἄτομος/átomos, α-τεμνω, which means uncuttable, something that cannot be divided further. The philosophical concept that matter might be composed of discrete units that cannot be further divided has been around for millennia. However, empirical proof and the universally acceptance of the existence of atoms didn’t occur until the early 1900’s.
As the science of chemistry matured, experimental evidence for the existence of atoms came from the law of multiple proportions. When two or more elements combined to form a compound, their masses are always in a fixed and definite ratio. For example, the mass ratio of nitrogen to oxygen in nitric oxide is seven eights. Ammonia has a hydrogen to nitrogen mass ratio of three fourteenths. The fact that elemental masses combined in simple fractions implies that all elemental mass stems from a common source. In principle, the atomic mass situation is analogous to the above example of Roman mass units. The Roman pound and ounce were both defined in terms of different sized collections of carob seeds, and consequently, the two mass units were related to each other through a simple fraction. Comparatively, since all of the atomic masses are related to each other through simple fractions, then perhaps the atomic masses are just different sized collections of some common fundamental mass unit.
In 1805, the chemist John Dalton published his first table of relative atomic weights, listing six elements, hydrogen, oxygen, nitrogen, carbon, sulfur, and phosphorus, and assigning hydrogen an atomic weight of 1. And in 1815, the chemist William Prout concluded that the hydrogen atom was in fact the fundamental mass unit from which all other atomic masses were derived.

If Prout's hypothesis had proven accurate, then the abstract concept of mass, as we now know it, might have never evolved, since mass could always be defined in terms of amounts of the hydrogen atomic mass. Prout’s hypothesis; however, was found to be inaccurate in two major respects. First, further scientific advancements revealed the existence of smaller particles, such as electrons and quarks, whose masses are not related through simple fractions. And second, the elemental masses themselves were found to not be exact multiples of the hydrogen atom mass, but rather, they were near multiples. Einstein’s theory of relativity explained that when protons and neutrons come together to form an atomic nucleus, some of the mass of the nucleus is released in the form of binding energy. The more tightly bound the nucleus, the more energy is lost during formation and this binding energy loss causes the elemental masses to not be related through simple fractions.
Hydrogen, for example, with a single proton, has an atomic weight of 1.007825 u. The most abundant isotope of iron has 26 protons and 30 neutrons, so one might expect its atomic weight to be 56 times that of the hydrogen atom, but in fact, its atomic weight is only 55.9383 u, which is clearly not an integer multiple of 1.007825. Prout’s hypothesis was proven inaccurate in many respects, but the abstract concepts of atomic mass and amount continue to play an influential role, and the atomic mass unit continues to be the unit of choice for very small mass measurements. (Although, the definition of the atomic mass unit is no longer tied to the hydrogen atom).
When the French invented the metric system in the late 1700s, they used an amount to define their mass unit. The gram was originally defined to be equal in mass to the amount of pure water contained in a one milliliter container. This definition, however, was inadequate for the precision requirements of modern technology, and the metric kilogram was redefined in terms of a manmade physical object.
Gravitational Mass
Active Gravitational mass is a property of the mass of an object that produces a gravitational field in the space surrounding the object, and these gravitational fields govern large-scale structures in the universe. Gravitational fields hold the galaxies together. They cause clouds of gas and dust to coalesce into stars and planets. They provide the necessary pressure for nuclear fusion to occur within stars. And they determine the orbits of various objects within the solar system. Since gravitational effects are all around us, it is impossible to pin down the exact date when humans first discovered gravitational mass. However, it is possible to identify some of the significant steps towards our modern understanding of gravitational mass and its relationship to the other mass phenomena.
Aristotelian gravity
One issue that had puzzled scientists and philosophers was the relationship between an object’s weight and its free fall velocity. The Greek philosopher, Aristotle, had reasoned that every substance and every object has a natural place in the universe, and every object will try to achieve that position. Aristotle had reasoned that there were four terrestrial elements: earth, water, air, and fire (or heat). Aristotle supposed that the heavens were composed of a special "fifth element" called aether which was unlike the other elements. In Aristotle’s worldview, if a rock is released in a lake it will sink to the bottom because earth belongs at the bottom, beneath water, air, and fire. Rain falls from the sky because water belongs beneath air. Air bubbles rise to the surface of water because air belongs above water. And finally, heat from a fire will rise heavenward because heat belongs above earth, water, and air.
According to Aristotle, terrestrial forces result when an object is taken out of its natural position. When an earthly object (one composed of earth) is lifted, one feels a force of weight from the object trying to return to the earth, and upon being released the object returns to its natural position. In Aristotle’s worldview, falling objects are not compelled to move by an external force such as earth’s gravity, but rather, they move according to their own independent need to be in their natural positions. Aristotle further reasoned that a freely moving object would move at a constant speed. For example, raindrops appear to fall from the sky at a constant speed and air bubbles appear to rise in water at a constant speed. And Aristotle also supposed that the speed would be proportional to its weight but inversely proportional to the density of the medium through which the object was passing. For example, Aristotle believed that a rock would fall quickly through air but slowly through water, because water is denser than air, and that a heavy rock would fall quicker than a light one. Aristotle’s beliefs about celestial motion were somewhat analogous, but different, from his concepts of terrestrial motion. Aristotle believed that the planets, stars, and other celestial objects moved on their own without being acted upon by a gravitational force. However, celestial objects were unique in that terrestrial objects would eventually come to their natural position and then stop moving, whereas celestial objects continue to move forever and never come to rest.
For falling objects:
The observational limitations which prevented Aristotle and other early scientists and philosophers from accurately describing free fall motion were related but opposite to those which prevented early astronomers from accurately describing the solar system.

The early astronomers were limited by their inability to measure large distances. They could accurately measure the time periods required for each planet to complete a cycle, but they couldn’t measure the distances and paths followed to complete these orbits. Ironically, early scientist and philosophers could accurately measure the distance traveled when an object was dropped a short length, but they couldn’t accurately measure the short time periods involved in free fall motion. So early astronomers had failed to accurately characterize the solar system and early scientist and philosophers had failed to characterize earth's gravity. To overcome this limitation, Aristotle appears to have relied upon experiments with solid objects falling in water. However, water being dense and viscous had complicated the experimental results, thus confusing Aristotle’s comprehension of physical law.
Johannes Kepler was the first person to give an accurate description of the orbits of the planets, and by doing so; he was also the first person to describe gravitational mass. However, Kepler’s initial attempts to describe planetary orbits were inaccurate and were similar to the approaches taken by early Greek and Chinese philosophers. The Chinese, in the Wu Xing philosophy, had reasoned that there are five directions on a compass, five stages in a process (each with an associated element), and five planets visible to the naked eye. The Chinese therefore associated each planet with a particular direction and element. In a similar fashion, Greek philosophers approximately two thousands years prior to Kepler had proven that there were exactly five Platonic solids. The Greek philosophers had reasoned that each solid must be associated with a specific element, and Kepler further reasoned that perhaps the distances between the planetary orbits could be determined by placing Platonic solids inside of concentric spheres. This theory was somewhat successful, but didn’t agree with available astronomical data to the level of precision that Kepler desired.
Keplerian gravitational mass

English Name |
The Keplerian Planets | |||
---|---|---|---|---|
Semi-major axis | Sidereal orbital period | Mass of Sun | ||
Mercury | 0.387 099 AU | 0.240 842 sidereal year | ||
Venus | 0.723 332 AU | 0.615 187 sidereal year | ||
Mars | 1.523 662 AU | 1.880 816 sidereal year | ||
Jupiter | 5.203 363 AU | 11.861 776 sidereal year | ||
Saturn | 9.537 070 AU | 29.456 626 sidereal year |
After abandoning his Platonic solids model, Kepler began working with an array of traditional astronomical methods. In 1600 AD, Kepler sought employment with Tycho Brahe and consequently gained access to astronomical data of a higher precision than any previously available. Using Brahe’s precise observations of the planet Mars, Kepler proved that the traditional astronomical methods were inaccurate in their predictions, and he spent the next five years developing his own method for characterizing planetary motion. In Kepler’s final planetary model, he successfully described planetary orbits as following elliptical paths with the sun at a focal point of the ellipse. Kepler discovered that the square of the orbital period of a planet is directly proportional to the cube of the semi-major axis of its orbit; this is his third law of planetary motion. The concept of active gravitational mass is an immediate consequence of this law, since the square of the orbital period of a planet is directly proportional to the cube of the semi-major axis, then the ratio of these two values must be constant for all planets in the solar system. This constant ratio is a direct measure of the sun's active gravitational mass, it has units of distance cubed per time squared, and it is known as the standard gravitational parameter.
English Name |
The Galilean moons | |||
---|---|---|---|---|
Semi-major axis | Sidereal orbital period | Mass of Jupiter | ||
Io | 0.002 819 AU | 0.004 843 sidereal year | ||
Europa | 0.004 486 AU | 0.009 722 sidereal year | ||
Ganymede | 0.007 155 AU | 0.019 589 sidereal year | ||
Callisto | 0.012 585 AU | 0.045 694 sidereal year |
In 1609, Johannes Kepler published his three rules known as Kepler's laws of planetary motion, explaining how the planets follow elliptical orbits under the influence of the sun. In early January of 1610, Galileo Galilei observed four dim objects near Jupiter, which he mistook for stars. However, after a few days of observation, Galileo realized that these objects were in fact orbiting Jupiter. These four objects (later named the Galilean moons in honor of their discoverer) were the first celestial objects observed to orbit something other than the earth or sun. Galileo continued to observe these moons over the next eighteen months, and by the middle of 1611 he had obtained remarkably accurate estimates for their periods. Later, the semi-major axis of each moon was also estimated, thus allowing the gravitational mass of Jupiter to be determined from the orbits of its moons. The gravitational mass of Jupiter was found to be approximately a thousandth of the gravitational mass of the sun.
For orbiting planets:
Galilean gravitational field
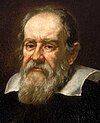
Galileo’s use of scientific instrumentation went beyond astronomical observations and the telescope. Sometime prior to 1638, Galileo had turned his attention to the phenomenon of objects falling under the influence of earth’s gravity, and he was actively attempting to characterize these motions. At the time, the relationship between gravity on earth (which caused objects to fall), and gravitational forces in heaven (which caused the planets to orbit) was unknown.

Galileo was not the first to investigate earth’s gravitational field, nor was he the first to accurately describe its fundamental characteristics. However, Galileo’s reliance on scientific experimentation to establish physical principles would have a profound effect on future generations of scientists. Galileo used a number of scientific experiments to characterize free fall motion. It is unclear if these were just hypothetical experiments used to illustrate a concept, or if they were real experiments performed by Galileo [3], but the results obtained from these experiments were both realistic and compelling. A biography by Galileo's pupil Vincenzo Viviani stated that Galileo had dropped balls of the same material, but different masses, from the Leaning Tower of Pisa to demonstrate that their time of descent was independent of their mass.[4] In support of this conclusion, Galileo had advanced the following theoretical argument: He asked if two bodies of different masses and different rates of fall are tied by a string, does the combined system fall faster because it is now more massive, or does the lighter body in its slower fall hold back the heavier body? The only convincing resolution to this question is that all bodies must fall at the same rate. [5]

A later experiment was described in Galileo’s Two New Sciences published in 1638. One of Galileo’s fictional characters, Salviati, describes an experiment using a bronze ball and a wooden ramp. The wooden ramp was "12 cubits long, half a cubit wide and three finger-breadths thick" with a straight, smooth, polished groove. The groove was lined with "parchment, also smooth and polished as possible". And into this groove was placed "a hard, smooth and very round bronze ball". The ramp was inclined at various angles to slow the acceleration enough so that the elapsed time could be measured. The ball was allowed to roll a known distance down the ramp, and the time taken for the ball to move the known distance was measured. The time was measured using a water clock described as follows:
- "a large vessel of water placed in an elevated position; to the bottom of this vessel was soldered a pipe of small diameter giving a thin jet of water, which we collected in a small glass during the time of each descent, whether for the whole length of the channel or for a part of its length; the water thus collected was weighed, after each descent, on a very accurate balance; the differences and ratios of these weights gave us the differences and ratios of the times, and this with such accuracy that although the operation was repeated many, many times, there was no appreciable discrepancy in the results.".[6]
This dialogue provides compelling and realistic solutions to the problems that had plagued earlier measurements. Previous experimenters were unable to measure the short time periods involved in free fall motion. Aristotle appears to have used water to slow the acceleration, but the frictional forces of the water had confused his experimental results. Galileo, on the other hand, by using a nearly frictionless inclined plane, was able to slow the acceleration without introducing additional frictional forces, and thus he was able to measure the fall times with his ingenious “water clock”. Galileo found that the distance traveled by an object in free fall was always proportional to the square of the elapsed time.
For falling objects:
Inertial and gravitational mass
Although inertial mass, passive gravitational mass and active gravitational mass are conceptually distinct, no experiment has ever unambiguously demonstrated any difference between them. In classical mechanics, Newton's third law implies that active and passive gravitational mass must always be identical (or at least proportional), but the classical theory offers no compelling reason why the gravitational mass has to equal the inertial mass. That it does is merely an empirical fact.
Albert Einstein developed his general theory of relativity starting from the assumption that this correspondence between inertial and (passive) gravitational mass is not accidental: that no experiment will ever detect a difference between them (the weak version of the equivalence principle). However, in the resulting theory gravitation is not a force and thus not subject to Newton's third law, so "the equality of inertial and active gravitational mass [...] remains as puzzling as ever".[7]
Inertial mass
- This section uses mathematical equations involving differential calculus.
Inertial mass is the mass of an object measured by its resistance to acceleration.
To understand what the inertial mass of a body is, one begins with classical mechanics and Newton's Laws of Motion. Later on, we will see how our classical definition of mass must be altered if we take into consideration the theory of special relativity, which is more accurate than classical mechanics. However, the implications of special relativity will not change the meaning of "mass" in any essential way.
According to Newton's second law, we say that a body has a mass m if, at any instant of time, it obeys the equation of motion
where F is the force acting on the body and a is the acceleration of the body.[note 2] For the moment, we will put aside the question of what "force acting on the body" actually means.
This equation illustrates how mass relates to the inertia of a body. Consider two objects with different masses. If we apply an identical force to each, the object with a bigger mass will experience a smaller acceleration, and the object with a smaller mass will experience a bigger acceleration. We might say that the larger mass exerts a greater "resistance" to changing its state of motion in response to the force.
However, this notion of applying "identical" forces to different objects brings us back to the fact that we have not really defined what a force is. We can sidestep this difficulty with the help of Newton's third law, which states that if one object exerts a force on a second object, it will experience an equal and opposite force. To be precise, suppose we have two objects A and B, with constant inertial masses mA and mB. We isolate the two objects from all other physical influences, so that the only forces present are the force exerted on A by B, which we denote FAB, and the force exerted on B by A, which we denote FBA. Newton's second law states that
where aA and aB are the accelerations of A and B, respectively. Suppose that these accelerations are non-zero, so that the forces between the two objects are non-zero. This occurs, for example, if the two objects are in the process of colliding with one another. Newton's third law then states that
and thus
Note that our requirement that aA be non-zero ensures that the fraction is well-defined.
This is, in principle, how we would measure the inertial mass of an object. We choose a "reference" object and define its mass mB as (say) 1 kilogram. Then we can measure the mass of any other object in the universe by colliding it with the reference object and measuring the accelerations.
Newtonian Gravitational mass
The Newtonian concept of gravitational mass rests on Newton's law of gravitation. Let us suppose we have two objects A and B, separated by a distance rAB. The law of gravitation states that if A and B have gravitational masses MA and MB respectively, then each object exerts a gravitational force on the other, of magnitude
where G is the universal gravitational constant. The above statement may be reformulated in the following way: if g is the acceleration of a reference mass at a given location in a gravitational field, then the gravitational force on an object with gravitational mass M is
This is the basis by which masses are determined by weighing. In simple spring scales, for example, the force F is proportional to the displacement of the spring beneath the weighing pan, as per Hooke's law, and the scales are calibrated to take g into account, allowing the mass M to be read off. A balance measures gravitational mass; only the spring scale measures weight.
Equivalence of inertial and gravitational masses
The equivalence of inertial and gravitational masses is sometimes referred to as the Galilean equivalence principle or weak equivalence principle. The most important consequence of this equivalence principle applies to freely falling objects. Suppose we have an object with inertial and gravitational masses m and M respectively. If the only force acting on the object comes from a gravitational field g, combining Newton's second law and the gravitational law yields the acceleration
This says that the ratio of gravitational to inertial mass of any object is equal to some constant K if and only if all objects fall at the same rate in a given gravitational field. This phenomenon is referred to as the 'universality of free-fall'. (In addition, the constant K can be taken to be 1 by defining our units appropriately.)
The first experiments demonstrating the universality of free-fall were conducted by Galileo. It is commonly stated that Galileo obtained his results by dropping objects from the Leaning Tower of Pisa, but this is most likely apocryphal; actually, he performed his experiments with balls rolling down inclined planes. Increasingly precise experiments have been performed, such as those performed by Loránd Eötvös, using the torsion balance pendulum, in 1889. As of 2008[update], no deviation from universality, and thus from Galilean equivalence, has ever been found, at least to the accuracy 10-12. More precise experimental efforts are still being carried out.
The universality of free-fall only applies to systems in which gravity is the only acting force. All other forces, especially friction and air resistance, must be absent or at least negligible. For example, if a hammer and a feather are dropped from the same height through the air on Earth, the feather will take much longer to reach the ground; the feather is not really in free-fall because the force of air resistance upwards against the feather is comparable to the downward force of gravity. On the other hand, if the experiment is performed in a vacuum, in which there is no air resistance, the hammer and the feather should hit the ground at exactly the same time (assuming the acceleration of both objects towards each other, and of the ground towards both objects, for its own part, is negligible). This can easily be done in a high school laboratory by dropping the objects in transparent tubes that have the air removed with a vacuum pump. It is even more dramatic when done in an environment that naturally has a vacuum, as David Scott did on the surface of the Moon during Apollo 15.
A stronger version of the equivalence principle, known as the Einstein equivalence principle or the strong equivalence principle, lies at the heart of the general theory of relativity. Einstein's equivalence principle states that within sufficiently small regions of space-time, it is impossible to distinguish between a uniform acceleration and a uniform gravitational field. Thus, the theory postulates that the force acting on a massive object caused by a gravitational field is a result of the object's tendency to move in a straght line (in other words its inertia) and should therefore be a function of its inertial mass and the strength of the gravitational field.
Mass and energy in relativity
The term mass in special relativity usually refers to the rest mass of the object, which is the Newtonian mass as measured by an observer moving along with the object. The invariant mass is another name for the rest mass of single particles. However, the more general invariant mass (calculated with a more complicated formula) may also be applied to systems of particles in relative motion, and because of this, is usually reserved for systems which consist of widely separated high-energy particles. The invariant mass of systems is the same for all observers and inertial frames, and cannot be destroyed, and is thus conserved, so long as the system is closed. In this case, "closure" implies that an idealized boundary is drawn around the system, and no mass/energy is allowed across it. In as much as energy is conserved in closed systems in relativity, the relativistic definition(s) of mass are quantities which are conserved; also, they do not change over time, even as some types of particles are converted to others.
In bound systems, the binding energy must (often) be subtracted from the mass of the unbound system, simply because this energy has mass, and this mass is subtracted from the system when it is given off, at the time it is bound. Mass is not conserved in this process because the system is not closed during the binding process. A familiar example is the binding energy of atomic nuclei, which appears as other types of energy (such as gamma rays) when the nuclei are formed, and (after being given off) results in nuclides which have less mass than the free particles (nucleons) of which they are composed.
The term relativistic mass is also used, and this is the total quantity of energy in a body or system (divided by c2). The relativistic mass (of a body or system of bodies) includes a contribution from the kinetic energy of the body, and is larger the faster the body moves, so unlike the invariant mass, the relativistic mass depends on the observer's frame of reference. However, for given single frames of reference and for closed systems, the relativistic mass is also a conserved quantity.
Because the relativistic mass is proportional to the energy, it has gradually fallen into disuse among physicists.[8] There is disagreement over whether the concept remains pedagogically useful.[9][10]
For a discussion of mass in general relativity, see mass in general relativity.
Notes
- ^ Since the Avogadro constant NA is defined as the number of atoms in 12 g of carbon-12, it follows that 1 u is exactly 1/(103 NA) kg.
- ^ Newton's second law is valid only for bodies of constant mass.
References
- R.V. Eötvös et al., Ann. Phys. (Leipzig) 68 11 (1922)
- E.F. Taylor, J.A. Wheeler (1992). Spacetime Physics. New York: W.H. Freeman. ISBN 0-7167-2327-1.
- ^ "massive, adj." OED Online. June 2009. Oxford University Press. 24 Sep. 2009 <http://dictionary.oed.com/cgi/entry/00302972>.
- ^ W. Rindler (2006). op. cit.. Oxford: Oxford Univ. Press. p. 16; Section 1.12. ISBN 0198567316.
- ^ Stillman Drake (1973). "Galileo's Discovery of the Law of Free Fall". Scientific American v. 228, #5, pp. 84-92.
- ^ Drake (1978, pp.19,20). At the time when Viviani asserts that the experiment took place, Galileo had not yet formulated the final version of his law of free fall. He had, however, formulated an earlier version which predicted that bodies of the same material falling through the same medium would fall at the same speed (Drake, 1978, p.20).
- ^ Galilei, Galileo (1632), Dialogue Concerning the Two Chief World Systems
- ^ Galileo 1638 Discorsi e dimostrazioni matematiche, intorno à due nuove scienze 213, Leida, Appresso gli Elsevirii (Leiden: Louis Elsevier), or Mathematical discourses and demonstrations, relating to Two New Sciences, English translation by Henry Crew and Alfonso de Salvio 1914. Section 213 is reprinted on pages 534-535 of On the Shoulders of Giants: The Great Works of Physics and Astronomy (works by Copernicus, Kepler, Galileo, Newton, and Einstein). Stephen Hawking, ed. 2002 ISBN 0-7624-1348-4
- ^ W. Rindler (2006). op. cit.. Oxford: Oxford Univ. Press. p. 22; end of Section 1.14. ISBN 0198567316.
- ^
G. Oas (2005). "On the Abuse and Use of Relativistic Mass". arXiv:physics/0504110.
{{cite arXiv}}
:|class=
ignored (help) - ^
L.B. Okun (1989). "The Concept of Mass" ([dead link] – Scholar search). Physics Today. 42 (6): 31–36. doi:10.1063/1.881171.
{{cite journal}}
: External link in
(help)|format=
- ^ T. R. Sandin (1991). "In defense of relativistic mass". American Journal of Physics. 59 (11): 1032. doi:10.1119/1.16642.
External links
- Stanford Encyclopedia of Philosophy: "The Equivalence of Mass and Energy" by Francisco Flores.
- "The Mysteries of Mass," Scientific American, July 2005.
- Usenet Physics FAQ by John Baez:
- "The Origin of Mass and the Feebleness of Gravity." Video of lecture by the Nobel Laureate Frank Wilczek.
- Okun, L. B., "Photons, Clocks, Gravity and the Concept of Mass." Slides for a talk.
- The Apollo 15 Hammer-Feather Drop.
- Online mass units conversion.
- Jerome, Jacky (2009) "Mass and Gravity."