Marine primary production: Difference between revisions
Epipelagic (talk | contribs) |
Epipelagic (talk | contribs) from marine microorganism |
||
Line 60: | Line 60: | ||
Most chloroplasts can probably be traced back to a single [[endosymbiotic event]], when a cyanobacterium was engulfed by the eukaryote. Despite this, chloroplasts can be found in an extremely wide set of organisms, some not even directly related to each other—a consequence of many [[secondary endosymbiotic events|secondary]] and even [[tertiary endosymbiotic events]]. |
Most chloroplasts can probably be traced back to a single [[endosymbiotic event]], when a cyanobacterium was engulfed by the eukaryote. Despite this, chloroplasts can be found in an extremely wide set of organisms, some not even directly related to each other—a consequence of many [[secondary endosymbiotic events|secondary]] and even [[tertiary endosymbiotic events]]. |
||
====Microbial rhodopsin==== |
|||
[[File:Model of the energy generating mechanism in marine bacteria.jpg|thumb|upright=1.9| {{center|'''Model of the energy generating mechanism in marine bacteria'''}} (1) When sunlight strikes a rhodopsin molecule<br /> (2) it changes its configuration so a proton is expelled from the cell<br /> (3) the chemical potential causes the proton to flow back to the cell<br /> (4) thus generating energy<br /> (5) in the form of [[adenosine triphosphate]].<ref>{{cite journal |last=DeLong |first=E.F. |last2=Beja |first2=O. |year=2010 |title=The light-driven proton pump proteorhodopsin enhances bacterial survival during tough times |journal=PLOS Biology |volume=8 |issue=4 |id=e1000359 |doi=10.1371/journal.pbio.1000359 |pages=e1000359 |pmid=20436957 |pmc=2860490}}</ref>]] |
|||
{{main|Microbial rhodopsin}} |
|||
Phototrophic metabolism relies on one of three energy-converting pigments: [[chlorophyll]], [[bacteriochlorophyll]], and [[retinal]]. Retinal is the [[chromophore]] found in [[rhodopsin]]s. The significance of chlorophyll in converting light energy has been written about for decades, but phototrophy based on retinal pigments is just beginning to be studied.<ref name=Gómez-Consarnau2019>{{cite journal | last1 = Gómez-Consarnau | first1 = L. | last2 = Raven | first2 = J.A. | last3 = Levine | first3 = N.M. | last4 = Cutter | first4 = L.S. | last5 = Wang | first5 = D. | last6 = Seegers | first6 = B. | last7 = Arístegui | first7 = J. | last8 = Fuhrman | first8 = J.A. | last9 = Gasol | first9 = J.M. | last10 = Sañudo-Wilhelmy | first10 = S.A. | year = 2019 | title = Microbial rhodopsins are major contributors to the solar energy captured in the sea | url = | journal = Science Advances | volume = 5| issue = 8| page = eaaw8855| doi = 10.1126/sciadv.aaw8855 | pmid = 31457093 | pmc = 6685716 | bibcode = 2019SciA....5.8855G | doi-access = free }}</ref> |
|||
[[File:Salt ponds SF Bay (dro!d).jpg|thumb|left|upright=0.80| [[Halobacteria]] in [[salt evaporation ponds]] coloured purple by [[bacteriorhodopsin]]<ref name="Oren2002">{{cite journal|last1=Oren|first1=Aharon|title=Molecular ecology of extremely halophilic Archaea and Bacteria|journal=FEMS Microbiology Ecology|volume=39|issue=1|year=2002|pages=1–7|url=http://femsec.oxfordjournals.org/content/39/1/1.full|issn=0168-6496|doi=10.1111/j.1574-6941.2002.tb00900.x|pmid=19709178|doi-access=free}}</ref>]] |
|||
In 2000 a team of microbiologists led by [[Edward DeLong]] made a crucial discovery in the understanding of the marine carbon and energy cycles. They discovered a gene in several species of bacteria<ref>{{cite journal | last1 = Béja | first1 = O. | last2 = Aravind | first2 = L. | last3 = Koonin | first3 = E.V. | last4 = Suzuki | first4 = M.T. | last5 = Hadd | first5 = A. | last6 = Nguyen | first6 = L.P. | last7 = Jovanovich | first7 = S.B. | last8 = Gates | first8 = C.M. | last9 = Feldman | first9 = R.A. | last10 = Spudich | first10 = J.L. | last11 = Spudich | first11 = E.N. | year = 2000 | title = Bacterial rhodopsin: evidence for a new type of phototrophy in the sea | url = https://semanticscholar.org/paper/d3dabf678450fbb8b518c1e963d24390f2942920| journal = Science | volume = 289 | issue = 5486| pages = 1902–1906 | doi = 10.1126/science.289.5486.1902 | pmid=10988064| bibcode = 2000Sci...289.1902B }}</ref><ref name="AcASM">{{cite web |url=http://academy.asm.org/index.php/news-views/interviews-with-fellows/366-ed-delong |title=Interviews with Fellows: Ed Delong |work=[[American Academy of Microbiology]] |accessdate=2 July 2016 |archive-url=https://web.archive.org/web/20160807061814/http://academy.asm.org/index.php/news-views/interviews-with-fellows/366-ed-delong |archive-date=7 August 2016 |url-status=dead }}</ref> responsible for production of the protein [[rhodopsin]], previously unheard of in bacteria. These proteins found in the cell membranes are capable of converting light energy to biochemical energy due to a change in configuration of the rhodopsin molecule as sunlight strikes it, causing the pumping of a [[proton]] from inside out and a subsequent inflow that generates the energy.<ref name="PopSci">''Bacteria with Batteries'', Popular Science, January 2001, Page 55.</ref> The archaeal-like rhodopsins have subsequently been found among different taxa, protists as well as in bacteria and archaea, though they are rare in complex [[multicellular organism]]s.<ref name=Béja2000>{{cite journal | last1 = Béja | first1 = O. | last2 = Aravind | first2 = L. | last3 = Koonin | first3 = E.V. | last4 = Suzuki | first4 = M.T. | last5 = Hadd | first5 = A. | last6 = Nguyen | first6 = L.P. | last7 = Jovanovich | first7 = S.B. | last8 = Gates | first8 = C.M. | last9 = Feldman | first9 = R.A. | last10 = Spudich | first10 = J.L. | last11 = Spudich | first11 = E.N. | year = 2000 | title = Bacterial rhodopsin: evidence for a new type of phototrophy in the sea | url = | journal = Science | volume = 289 | issue = 5486| pages = 1902–1906 | doi = 10.1126/science.289.5486.1902 | pmid = 10988064 | bibcode = 2000Sci...289.1902B }}</ref><ref name="BoeufAudic2015">{{cite journal|last1=Boeuf|first1=Dominique|last2=Audic|first2=Stéphane|last3=Brillet-Guéguen|first3=Loraine|last4=Caron|first4=Christophe|last5=Jeanthon|first5=Christian|title=MicRhoDE: a curated database for the analysis of microbial rhodopsin diversity and evolution|journal=Database|volume=2015|year=2015|pages=bav080|url=http://database.oxfordjournals.org/content/2015/bav080.full|issn=1758-0463|doi=10.1093/database/bav080|pmid=26286928|pmc=4539915}}</ref><ref name="YawoKandori2015">{{cite book|last1=Yawo|first1=Hiromu|last2=Kandori|first2=Hideki|last3=Koizumi|first3=Amane|title=Optogenetics: Light-Sensing Proteins and Their Applications|url=https://books.google.com/books?id=5M3WCQAAQBAJ&pg=PA3|accessdate=30 September 2015|date=5 June 2015|publisher=Springer|isbn=978-4-431-55516-2|pages=3–4}}</ref> |
|||
Research in 2019 shows these "sun-snatching bacteria" are more widespread than previously thought and could change how oceans are affected by global warming. "The findings break from the traditional interpretation of marine ecology found in textbooks, which states that nearly all sunlight in the ocean is captured by chlorophyll in algae. Instead, rhodopsin-equipped bacteria function like hybrid cars, powered by organic matter when available — as most bacteria are — and by sunlight when nutrients are scarce."<ref>[https://pressroom.usc.edu/a-tiny-marine-microbe-could-play-a-big-role-in-climate-change/ A tiny marine microbe could play a big role in climate change] ''University of Southern California'', Press Room, 8 August 2019.</ref><ref name=Gómez-Consarnau2019 /> |
|||
There is an [[astrobiology|astrobiological]] conjecture called the [[Purple Earth hypothesis]] which surmises that original life forms on Earth were retinal-based rather than chlorophyll-based, which would have made the Earth appear purple instead of green.<ref>{{Cite journal|last=DasSarma|first=Shiladitya|last2=Schwieterman|first2=Edward W.|date=11 October 2018|title=Early evolution of purple retinal pigments on Earth and implications for exoplanet biosignatures|url=https://www.cambridge.org/core/journals/international-journal-of-astrobiology/article/early-evolution-of-purple-retinal-pigments-on-earth-and-implications-for-exoplanet-biosignatures/63A1AD8AF544BEEF4C6D4A2D53130327|journal=International Journal of Astrobiology|pages=1–10|doi=10.1017/S1473550418000423|issn=1473-5504|arxiv=1810.05150|df=dmy-all|bibcode=2018arXiv181005150D}}</ref><ref>{{cite journal|last1=Sparks|first1=William B. |last2=DasSarma|first2=S. |last3=Reid|first3=I. N. |title=Evolutionary Competition Between Primitive Photosynthetic Systems: Existence of an early purple Earth? |journal=American Astronomical Society Meeting Abstracts |bibcode=2006AAS...209.0605S|volume=38|page=901|date=December 2006|df=dmy-all}}</ref> |
|||
{{clear}} |
|||
==Marine algae== |
==Marine algae== |
Revision as of 03:14, 12 May 2020

Part of a series of overviews on |
Marine life |
---|
![]() |
Marine primary production is the chemical synthesis in the ocean of organic compounds from atmospheric or dissolved carbon dioxide. It principally occurs through the process of photosynthesis, which uses light as its source of energy, but it also occurs through chemosynthesis, which uses the oxidation or reduction of inorganic chemical compounds as its source of energy. Almost all life on Earth relies directly or indirectly on primary production. The organisms responsible for primary production are called primary producers or autotrophs.
Marine primary production is generated mainly by a diverse collection of algae that, together with cyanobacteria form the principal primary producers at base of the ocean food chain. Marine primary producers are important because they underpin almost all marine animal life by generating most of the oxygen and food that marine animals need to exist. Some marine primary producers are also ecosystem engineers which change the environment and provide habitats for other marine life.
Primary production in the ocean can be contrasted with primary production on land. Globally the ocean and the land each produce about the same amount of primary production, but in the ocean primary production comes mainly from cyanobacteria and algae, while on land it comes mainly from vascular plants.
Marine algae includes the largely invisible and often unicellular microalgae, which together with cyanobacteria form the ocean phytoplankton, as well as the larger, more visible and complex multicellular macroalgae commonly called seaweed. Seaweeds are found along coastal areas, living on the floor of continental shelves and washed up in intertidal zones. Some seaweeds drift with plankton in the sunlit surface waters (epipelagic zone) of the open ocean.
Back in the Silurian, some phytoplankton evolved into red, brown and green algae. These algae then invaded the land and started evolving into the land plants we know today. Later in the Cretaceous some of these land plants returned to the sea as mangroves and seagrasses. These are found along coasts in intertidal regions and in the brackish water of estuaries. In addition, some seagrasses, like seaweeds, can be found at depths up to 50 metres on both soft and hard bottoms of the continental shelf.
Primary producers
Primary producers are the autotroph organisms that make their own food instead of eating other organisms. This means primary producers become the starting point in the food chain for heterotroph organisms that do eat other organisms. Some marine primary producers are specialised bacteria and archaea which are chemotrophs, making their own food by gathering around hydrothermal vents and cold seeps and using chemosynthesis. However most marine primary production comes from organisms which use photosynthesis on the carbon dioxide dissolved in the water. This process uses energy from sunlight to convert water and carbon dioxide[2]: 186–187 into sugars that can be used both as a source of chemical energy and of organic molecules that are used in the structural components of cells.[2]: 1242 Marine primary producers are important because they underpin almost all marine animal life by generating most of the oxygen and food that provide other organisms with the chemical energy they need to exist.
The principal marine primary producers are cyanobacteria, algae and marine plants. The oxygen released as a by-product of photosynthesis is needed by nearly all living things to carry out cellular respiration. In addition, primary producers are influential in the global carbon and water cycles. They stabilize coastal areas and can provide habitats for marine animals. The term division has been traditionally used instead of phylum when discussing primary producers, although the International Code of Nomenclature for algae, fungi, and plants now accepts the terms as equivalent.[3]
In a reversal of the pattern on land, in the oceans, almost all photosynthesis is performed by algae and cyanobacteria, with a small fraction contributed by vascular plants and other groups. Algae encompass a diverse range of organisms, ranging from single floating cells to attached seaweeds. They include photoautotrophs from a variety of groups. Eubacteria are important photosynthetizers in both oceanic and terrestrial ecosystems, and while some archaea are phototrophic, none are known to utilise oxygen-evolving photosynthesis.[4] A number of eukaryotes are significant contributors to primary production in the ocean, including green algae, brown algae and red algae, and a diverse group of unicellular groups. Vascular plants are also represented in the ocean by groups such as the seagrasses.
Unlike terrestrial ecosystems, the majority of primary production in the ocean is performed by free-living microscopic organisms called phytoplankton. Larger autotrophs, such as the seagrasses and macroalgae (seaweeds) are generally confined to the littoral zone and adjacent shallow waters, where they can attach to the underlying substrate but still be within the photic zone. There are exceptions, such as Sargassum, but the vast majority of free-floating production takes place within microscopic organisms.
The factors limiting primary production in the ocean are also very different from those on land. The availability of water, obviously, is not an issue (though its salinity can be). Similarly, temperature, while affecting metabolic rates (see Q10), ranges less widely in the ocean than on land because the heat capacity of seawater buffers temperature changes, and the formation of sea ice insulates it at lower temperatures. However, the availability of light, the source of energy for photosynthesis, and mineral nutrients, the building blocks for new growth, play crucial roles in regulating primary production in the ocean.[5] Available Earth System Models suggest that ongoing ocean bio-geochemical changes could trigger reductions in ocean NPP between 3% and 10% of current values depending on the emissions scenario.[6]
Cyanobacteria

Cyanobacteria are a phylum (division) of bacteria which range from unicellular to filamentous and include colonial species. They are found almost everywhere on earth: in damp soil, in both freshwater and marine environments, and even on Antarctic rocks.[7] In particular, some species occur as drifting cells floating in the ocean, and as such were amongst the first of the phytoplankton.
The first primary producers that used photosynthesis were oceanic cyanobacteria about 2.3 billion years ago.[8][9] The release of molecular oxygen by cyanobacteria as a by-product of photosynthesis induced global changes in the Earth's environment. Because oxygen was toxic to most life on Earth at the time, this led to the near-extinction of oxygen-intolerant organisms, a dramatic change which redirected the evolution of the major animal and plant species.[10]
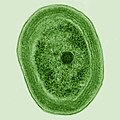
The tiny marine cyanobacterium Prochlorococcus, discovered in 1986, forms today part of the base of the ocean food chain and accounts for more than half the photosynthesis of the open ocean[11] and an estimated 20% of the oxygen in the Earth's atmosphere.[12] It is possibly the most plentiful genus on Earth: a single millilitre of surface seawater may contain 100,000 cells or more.[13]
Originally, biologists thought cyanobacteria was algae, and referred to it as "blue-green algae". The more recent view is that cyanobacteria is a bacteria, and hence is not even in the same Kingdom as algae. Most authorities exclude all prokaryotes, and hence cyanobacteria from the definition of algae.[14][15]
Biological pigments
Biological pigments are any coloured material in plant or animal cells. All biological pigments selectively absorb certain wavelengths of light while reflecting others.[16][17] The primary function of pigments in plants is photosynthesis, which uses the green pigment chlorophyll and several colorful pigments that absorb as much light energy as possible. Chlorophyll is the primary pigment in plants; it is a chlorin that absorbs yellow and blue wavelengths of light while reflecting green. It is the presence and relative abundance of chlorophyll that gives plants their green color. Green algae and plants possess two forms of this pigment: chlorophyll a and chlorophyll b. Kelps, diatoms, and other photosynthetic heterokonts contain chlorophyll c instead of b, while red algae possess only chlorophyll a. All chlorophylls serve as the primary means plants use to intercept light in order to fuel photosynthesis.
Chloroplasts
Chloroplasts (from the Greek chloros for green, and plastes for "the one who forms"[18]) are organelles that conduct photosynthesis, where the photosynthetic pigment chlorophyll captures the energy from sunlight, converts it, and stores it in the energy-storage molecules while freeing oxygen from water in plant and algal cells. They then use the stored energy to make organic molecules from carbon dioxide in a process known as the Calvin cycle.

A chloroplast is a type of organelle known as a plastid, characterized by its two membranes and a high concentration of chlorophyll. They are highly dynamic—they circulate and are moved around within plant cells, and occasionally pinch in two to reproduce. Their behavior is strongly influenced by environmental factors like light color and intensity. Chloroplasts, like mitochondria, contain their own DNA, which is thought to be inherited from their ancestor—a photosynthetic cyanobacterium that was engulfed by an early eukaryotic cell.[20] Chloroplasts cannot be made by the plant cell and must be inherited by each daughter cell during cell division.
Most chloroplasts can probably be traced back to a single endosymbiotic event, when a cyanobacterium was engulfed by the eukaryote. Despite this, chloroplasts can be found in an extremely wide set of organisms, some not even directly related to each other—a consequence of many secondary and even tertiary endosymbiotic events.
Microbial rhodopsin

(2) it changes its configuration so a proton is expelled from the cell
(3) the chemical potential causes the proton to flow back to the cell
(4) thus generating energy
(5) in the form of adenosine triphosphate.[21]
Phototrophic metabolism relies on one of three energy-converting pigments: chlorophyll, bacteriochlorophyll, and retinal. Retinal is the chromophore found in rhodopsins. The significance of chlorophyll in converting light energy has been written about for decades, but phototrophy based on retinal pigments is just beginning to be studied.[22]

In 2000 a team of microbiologists led by Edward DeLong made a crucial discovery in the understanding of the marine carbon and energy cycles. They discovered a gene in several species of bacteria[24][25] responsible for production of the protein rhodopsin, previously unheard of in bacteria. These proteins found in the cell membranes are capable of converting light energy to biochemical energy due to a change in configuration of the rhodopsin molecule as sunlight strikes it, causing the pumping of a proton from inside out and a subsequent inflow that generates the energy.[26] The archaeal-like rhodopsins have subsequently been found among different taxa, protists as well as in bacteria and archaea, though they are rare in complex multicellular organisms.[27][28][29]
Research in 2019 shows these "sun-snatching bacteria" are more widespread than previously thought and could change how oceans are affected by global warming. "The findings break from the traditional interpretation of marine ecology found in textbooks, which states that nearly all sunlight in the ocean is captured by chlorophyll in algae. Instead, rhodopsin-equipped bacteria function like hybrid cars, powered by organic matter when available — as most bacteria are — and by sunlight when nutrients are scarce."[30][22]
There is an astrobiological conjecture called the Purple Earth hypothesis which surmises that original life forms on Earth were retinal-based rather than chlorophyll-based, which would have made the Earth appear purple instead of green.[31][32]
Marine algae
Algae is an informal term for a widespread and diverse group of photosynthetic eukaryotic organisms which are not necessarily closely related and are thus polyphyletic. Unlike higher plants, algae lack roots, stems, or leaves. Marine algae mainly fall into five groups: green algae, red algae, brown algae, diatoms and dinoflagellates. There is also a phylum of unicellular flagellates called euglenophytes. However, these have few marine members.
Green algae
Green algae live most of their lives as single cells or are filamentous, while others form colonies made up from long chains of cells, or are highly differentiated macroscopic seaweeds. They form an informal group containing about 8,000 recognized species.[33]
Red algae
Modern red algae are mostly multicellular with differentiated cells and include many notable seaweeds.[34][35] As coralline algae, they play an important role in the ecology of coral reefs. They form a (disputed) phylum containing about 7,000 recognized species.[34]
-
Cyanidiophyceae colony, a class of unicellular red algae
-
The seaweed Porphyra umbilicalis
Brown algae
Brown algae are mostly multicellular and include many seaweeds, including kelp. They form a class containing about 2,000 recognized species.[36]
Diatoms
-
Diatoms are one of the most common types of phytoplankton
-
They are a major algae group generating about 20% of world oxygen production.[37]
Dinoflagellate
-
Dinoflagellates
-
Euglena mutabilis, a photosynthetic flagellate
Microalgae
Algae can also be classified by size as microalgae or macroalgae. Microalgae are the microscopic types of algae, not visible to the naked eye. They are mostly unicellular species which exist as individuals or in chains or groups, though some are multicellular. Microalgae are important components of the marine protists, as well as the marine phytoplankton. They are very diverse. It has been estimated there are 200,000-800,000 species of which about 50,000 species have been described.[39] Depending on the species, their sizes range from a few micrometers (µm) to a few hundred micrometers. They are specially adapted to an environment dominated by viscous forces.
- Microalgae
-
Single-celled alga, Gephyrocapsa oceanica
-
Algae bloom of Emiliania huxleyi off the southern coast of England
-
Zooxanthellae is a photosynthetic algae that lives inside hosts like coral
Macroalgae

Macroalgae are the larger, multicellular and more visible types of algae, commonly called seaweeds. Seaweeds usually grow in shallow coastal waters where they are anchored to the seafloor by a holdfast. Seaweed that becomes adrift can wash up on beaches. Kelp is a large brown seaweed that forms large underwater forests covering about 25% of the world coastlines.[40] They are among the most productive and dynamic ecosystems on Earth.[41] Some Sargassum seaweeds are planktonic (free-floating). Like microalgae, macroalgae (seaweeds) are technically marine protists since they are not true plants.


- Macroalgae
-
Giant kelp is technically a protist since it is not a true plant, yet it is multicellular and can grow to 50 m
-
Sargassum seaweed is a brown alga with air bladders that help it float
-
Sargassum fish are camouflaged to live among drifting Sargassum seaweed
Marine plants

Back in the Silurian, some phytoplankton evolved into red, brown and green algae. These algae then invaded the land and started evolving into the land plants we know today. Later, in the Cretaceous, some of these land plants returned to the sea as mangroves and seagrasses.[43]
Marine plants can be found in intertidal zones and shallow waters, such as seagrasses like eelgrass and turtle grass, Thalassia. These plants have adapted to the high salinity of the ocean environment. Plant life can also flourish in the brackish waters of estuaries, where mangroves or cordgrass or beach grass beach grass might grow.
Mangroves
Mangroves provide important nursery habitats for marine life, acting as hiding and foraging places for larval and juvenile forms of larger fish and invertebrates. Based on satellite data, the total world area of mangrove forests was estimated in 2010 as 134,257 square kilometres (51,837 sq mi).[44][45]


- Spalding, M. (2010) World atlas of mangroves, Routledge. ISBN 9781849776608. doi:10.4324/9781849776608.
Seagrasses
Like mangroves, seagrasses provide important nursery habitats for larval and juvenile forms of larger fish and invertebrates. The total world area of seagrass meadows is more difficult to determine than mangrove forests, but was conservatively estimated in 2003 as 177,000 square kilometres (68,000 sq mi).[46]
-
Sea dragons camouflaged to look like floating seaweed live in kelp forests and seagrass meadows[47]
See also
- Algae
- Marine plants
- Evolutionary history of plants
- Plant evolution
- Timeline of plant evolution
- Evolution of photosynthesis
References
- ^ Chlorophyll NASA Earth Observatory. Accessed 30 November 2019.
- ^ a b Campbell, Neil A.; Reece, Jane B.; Urry, Lisa Andrea; Cain, Michael L.; Wasserman, Steven Alexander; Minorsky, Peter V.; Jackson, Robert Bradley (2008). Biology (8 ed.). San Francisco: Pearson – Benjamin Cummings. ISBN 978-0-321-54325-7.
{{cite book}}
: Invalid|ref=harv
(help) - ^ McNeill, J., ed. (2012). International Code of Nomenclature for algae, fungi, and plants (Melbourne Code), Adopted by the Eighteenth International Botanical Congress Melbourne, Australia, July 2011 (electronic ed.). International Association for Plant Taxonomy. Retrieved 2017-05-14.
{{cite book}}
: Unknown parameter|displayeditors=
ignored (|display-editors=
suggested) (help) - ^ Schäfer G, Engelhard M, Müller V (1 September 1999). "Bioenergetics of the Archaea". Microbiol. Mol. Biol. Rev. 63 (3): 570–620. doi:10.1128/MMBR.63.3.570-620.1999. PMC 103747. PMID 10477309.
- ^ Sigman, D.M.; Hain, M.P. (2012). "The Biological Productivity of the Ocean" (PDF). Nature Education Knowledge. 3 (6): 1–16. Retrieved 2015-06-01.
The deep chlorophyll maximum (DCM) occurs at the contact where there is adequate light for photosynthesis and yet significant nutrient supply from below.
- ^ Mora, C.; et al. (2013). "Biotic and Human Vulnerability to Projected Changes in Ocean Biogeochemistry over the 21st Century". PLoS Biology. 11 (10): e1001682. doi:10.1371/journal.pbio.1001682. PMC 3797030. PMID 24143135.
{{cite journal}}
: CS1 maint: unflagged free DOI (link) - ^ Walsh, Patrick J.; Smith, Sharon; Fleming, Lora; Solo-Gabriele, Helena; Gerwick, William H., eds. (2 September 2011). "Cyanobacteria and cyanobacterial toxins". Oceans and Human Health: Risks and Remedies from the Seas. Academic Press. pp. 271–296. ISBN 978-0-08-087782-2.
{{cite book}}
: Unknown parameter|name-list-format=
ignored (|name-list-style=
suggested) (help) - ^ "The Rise of Oxygen - Astrobiology Magazine". Astrobiology Magazine. 30 July 2003. Retrieved 2016-04-06.
- ^ Flannery, D. T.; R.M. Walter (2012). "Archean tufted microbial mats and the Great Oxidation Event: new insights into an ancient problem". Australian Journal of Earth Sciences. 59 (1): 1–11. Bibcode:2012AuJES..59....1F. doi:10.1080/08120099.2011.607849.
- ^ Rothschild, Lynn (September 2003). "Understand the evolutionary mechanisms and environmental limits of life". NASA. Archived from the original on 11 March 2012. Retrieved 13 July 2009.
- ^ Nadis S (December 2003). "The cells that rule the seas" (PDF). Scientific American. 289 (6): 52–3. Bibcode:2003SciAm.289f..52N. doi:10.1038/scientificamerican1203-52. PMID 14631732. Archived from the original (PDF) on 2014-04-19. Retrieved 2019-07-11.
- ^ "The Most Important Microbe You've Never Heard Of". npr.org.
- ^ Flombaum, P.; Gallegos, J. L.; Gordillo, R. A.; Rincon, J.; Zabala, L. L.; Jiao, N.; Karl, D. M.; Li, W. K. W.; Lomas, M. W.; Veneziano, D.; Vera, C. S.; Vrugt, J. A.; Martiny, A. C. (2013). "Present and future global distributions of the marine Cyanobacteria Prochlorococcus and Synechococcus". Proceedings of the National Academy of Sciences. 110 (24): 9824–9829. Bibcode:2013PNAS..110.9824F. doi:10.1073/pnas.1307701110. PMC 3683724. PMID 23703908.
- ^ Nabors, Murray W. (2004). Introduction to Botany. San Francisco, CA: Pearson Education, Inc. ISBN 978-0-8053-4416-5.
- ^ Allaby, M., ed. (1992). "Algae". The Concise Dictionary of Botany. Oxford: Oxford University Press.
- ^ Grotewold, E. (2006). "The Genetics and Biochemistry of Floral Pigments". Annual Review of Plant Biology. 57: 761–780. doi:10.1146/annurev.arplant.57.032905.105248.
- ^ Lee, DW (2007) Nature's palette - the science of plant color. University of Chicago Press
- ^ "chloroplast". Online Etymology Dictionary.
- ^ Patrick J. Keeling (2004). "Diversity and evolutionary history of plastids and their hosts". American Journal of Botany. 91 (10): 1481–1493. doi:10.3732/ajb.91.10.1481. PMID 21652304.
- ^ Basic Biology (18 March 2016). "Bacteria".
- ^ DeLong, E.F.; Beja, O. (2010). "The light-driven proton pump proteorhodopsin enhances bacterial survival during tough times". PLOS Biology. 8 (4): e1000359. doi:10.1371/journal.pbio.1000359. PMC 2860490. PMID 20436957. e1000359.
{{cite journal}}
: CS1 maint: unflagged free DOI (link) - ^ a b Gómez-Consarnau, L.; Raven, J.A.; Levine, N.M.; Cutter, L.S.; Wang, D.; Seegers, B.; Arístegui, J.; Fuhrman, J.A.; Gasol, J.M.; Sañudo-Wilhelmy, S.A. (2019). "Microbial rhodopsins are major contributors to the solar energy captured in the sea". Science Advances. 5 (8): eaaw8855. Bibcode:2019SciA....5.8855G. doi:10.1126/sciadv.aaw8855. PMC 6685716. PMID 31457093.
- ^ Oren, Aharon (2002). "Molecular ecology of extremely halophilic Archaea and Bacteria". FEMS Microbiology Ecology. 39 (1): 1–7. doi:10.1111/j.1574-6941.2002.tb00900.x. ISSN 0168-6496. PMID 19709178.
- ^ Béja, O.; Aravind, L.; Koonin, E.V.; Suzuki, M.T.; Hadd, A.; Nguyen, L.P.; Jovanovich, S.B.; Gates, C.M.; Feldman, R.A.; Spudich, J.L.; Spudich, E.N. (2000). "Bacterial rhodopsin: evidence for a new type of phototrophy in the sea". Science. 289 (5486): 1902–1906. Bibcode:2000Sci...289.1902B. doi:10.1126/science.289.5486.1902. PMID 10988064.
- ^ "Interviews with Fellows: Ed Delong". American Academy of Microbiology. Archived from the original on 7 August 2016. Retrieved 2 July 2016.
- ^ Bacteria with Batteries, Popular Science, January 2001, Page 55.
- ^ Béja, O.; Aravind, L.; Koonin, E.V.; Suzuki, M.T.; Hadd, A.; Nguyen, L.P.; Jovanovich, S.B.; Gates, C.M.; Feldman, R.A.; Spudich, J.L.; Spudich, E.N. (2000). "Bacterial rhodopsin: evidence for a new type of phototrophy in the sea". Science. 289 (5486): 1902–1906. Bibcode:2000Sci...289.1902B. doi:10.1126/science.289.5486.1902. PMID 10988064.
- ^ Boeuf, Dominique; Audic, Stéphane; Brillet-Guéguen, Loraine; Caron, Christophe; Jeanthon, Christian (2015). "MicRhoDE: a curated database for the analysis of microbial rhodopsin diversity and evolution". Database. 2015: bav080. doi:10.1093/database/bav080. ISSN 1758-0463. PMC 4539915. PMID 26286928.
- ^ Yawo, Hiromu; Kandori, Hideki; Koizumi, Amane (5 June 2015). Optogenetics: Light-Sensing Proteins and Their Applications. Springer. pp. 3–4. ISBN 978-4-431-55516-2. Retrieved 30 September 2015.
- ^ A tiny marine microbe could play a big role in climate change University of Southern California, Press Room, 8 August 2019.
- ^ DasSarma, Shiladitya; Schwieterman, Edward W. (11 October 2018). "Early evolution of purple retinal pigments on Earth and implications for exoplanet biosignatures". International Journal of Astrobiology: 1–10. arXiv:1810.05150. Bibcode:2018arXiv181005150D. doi:10.1017/S1473550418000423. ISSN 1473-5504.
- ^ Sparks, William B.; DasSarma, S.; Reid, I. N. (December 2006). "Evolutionary Competition Between Primitive Photosynthetic Systems: Existence of an early purple Earth?". American Astronomical Society Meeting Abstracts. 38: 901. Bibcode:2006AAS...209.0605S.
- ^ Guiry MD (October 2012). "How many species of algae are there?". Journal of Phycology. 48 (5): 1057–63. doi:10.1111/j.1529-8817.2012.01222.x. PMID 27011267.
- ^ a b Guiry, M.D.; Guiry, G.M. (2016). "Algaebase". www.algaebase.org. Retrieved November 20, 2016.
- ^ D. Thomas (2002). Seaweeds. Life Series. Natural History Museum, London. ISBN 978-0-565-09175-0.
- ^ Hoek, Christiaan; den Hoeck, Hoeck Van; Mann, David; Jahns, H.M. (1995). Algae : an introduction to phycology. Cambridge University Press. p. 166. ISBN 9780521316873. OCLC 443576944.
- ^ The Air You're Breathing? A Diatom Made That
- ^ "More on Diatoms". University of California Museum of Paleontology. Archived from the original on 2012-10-04. Retrieved 2019-07-11.
- ^ Starckx, Senne (31 October 2012) A place in the sun - Algae is the crop of the future, according to researchers in Geel Flanders Today, Retrieved 8 December 2012
- ^ Kelp Forest - an overview | ScienceDirect Topics
- ^ Mann, K.H. 1973. Seaweeds: their productivity and strategy for growth. Science 182: 975-981.
- ^ Tunnell, John Wesley; Chávez, Ernesto A.; Withers, Kim (2007). Coral reefs of the southern Gulf of Mexico. Texas A&M University Press. p. 91. ISBN 978-1-58544-617-9.
- ^ Orth, R.J., Carruthers, T.J., Dennison, W.C., Duarte, C.M., Fourqurean, J.W., Heck, K.L., Hughes, A.R., Kendrick, G.A., Kenworthy, W.J., Olyarnik, S. and Short, F.T. (2006) "A global crisis for seagrass ecosystems". Bioscience, 56(12): pages 987–996. doi:10.1641/0006-3568(2006)56[987:AGCFSE2.0.CO;2]
- ^ Giri C, Ochieng E, Tieszen LL, Zhu Z, Singh A, Loveland T, et al. (2011) "Status and distribution of mangrove forests of the world using earth observation satellite data". Global Ecology and Biogeography, 20(1):154–159. doi:10.1111/j.1466-8238.2010.00584.x
- ^ Thomas, N., Lucas, R., Bunting, P., Hardy, A., Rosenqvist, A. and Simard, M. (2017) "Distribution and drivers of global mangrove forest change, 1996–2010". PLOS ONE, 12(6): e0179302. doi:10.1371/journal.pone.0179302
- ^ Short, F.T. and Frederick, T. (2003) World atlas of seagrasses, University of California Press, page 24. ISBN 9780520240476
- ^ Froese, Rainer; Pauly, Daniel (eds.) (2009). "Phycodurus eques" in FishBase. July 2009 version.