Physics: Difference between revisions
→History: cutting history section as mentioned on talk page. can we please discuss its length before reinserting it? |
Van helsing (talk | contribs) m I have no idea what is going on with this article the last couple of days but deleting the entire History section doesn’t sound like a good idea |
||
Line 34: | Line 34: | ||
[[Thermodynamics]] is the branch of physics that deals with the action of [[heat]] and the conversions from one to another of various forms of [[energy]]. Thermodynamics is particularly concerned with how these affect [[temperature]], [[pressure]], [[volume]], [[action (physics)|mechanical action]], [[entropy]], and [[Work (thermodynamics)|work]]. |
[[Thermodynamics]] is the branch of physics that deals with the action of [[heat]] and the conversions from one to another of various forms of [[energy]]. Thermodynamics is particularly concerned with how these affect [[temperature]], [[pressure]], [[volume]], [[action (physics)|mechanical action]], [[entropy]], and [[Work (thermodynamics)|work]]. |
||
===Statistical Mechanics=== |
|||
[[Statistical mechanics]] is the branch of physics that analyzes [[macroscopic]] [[thermodynamic system|systems]] by applying [[statistics|statistical principles]] to their microscopic constituents. It can be applied to calculate the thermodynamic properties of bulk materials from the properties of individual molecules, which is the basis of [[statistical thermodynamics]]. |
[[Statistical mechanics]] is the branch of physics that analyzes [[macroscopic]] [[thermodynamic system|systems]] by applying [[statistics|statistical principles]] to their microscopic constituents. It can be applied to calculate the thermodynamic properties of bulk materials from the properties of individual molecules, which is the basis of [[statistical thermodynamics]]. |
||
Line 78: | Line 79: | ||
[[Applied physics]] is physics that is intended for a particular technological or practical use, as for example in [[engineering]], as opposed to [[basic research]]. Applied physics is rooted in the fundamental truths and basic concepts of the physical sciences, but is concerned with the use of scientific principles in practical devices and systems, and in the application of physics in other areas of science. The approach is similar to that of [[applied mathematics]]. "Applied" is distinguished from "pure" by a subtle combination of factors such as the motivation and attitude of researchers and the nature of the relationship to the technology or science that may be affected by the work. [http://www.stanford.edu/dept/app-physics/general/] |
[[Applied physics]] is physics that is intended for a particular technological or practical use, as for example in [[engineering]], as opposed to [[basic research]]. Applied physics is rooted in the fundamental truths and basic concepts of the physical sciences, but is concerned with the use of scientific principles in practical devices and systems, and in the application of physics in other areas of science. The approach is similar to that of [[applied mathematics]]. "Applied" is distinguished from "pure" by a subtle combination of factors such as the motivation and attitude of researchers and the nature of the relationship to the technology or science that may be affected by the work. [http://www.stanford.edu/dept/app-physics/general/] |
||
== History == |
|||
{{main | History of physics}} |
|||
{{further | [[Famous physicists]], [[Nobel Prize in physics]]}} |
|||
[[Image:Francesco Hayez 001.jpg|thumb|150px|left|[[Aristotle]]]] |
|||
Since antiquity, people have tried to understand the workings of Nature and the behavior of [[matter]]: why unsupported objects drop to the ground, why different [[materials science|materials]] have different properties, and so forth. The character of the [[universe]] was also a mystery, for instance the [[earth]] and the behavior of celestial objects such as the [[sun]] and the [[moon]]. Several theories were proposed, most of which were incorrect, such as the earth orbiting the moon. These first theories were largely couched in [[philosophy|philosophical]] terms, and never verified by systematic experimental testing, as is popular today. The works of [[Ptolemy]] and [[Physics (Aristotle)|Aristotle]] were not always found to match everyday observations. There were exceptions and there are [[anachronism]]s - for example, [[Indian philosophy|Indian philosophers]] and [[Indian science and technology#Astronomy|astronomers]] gave many correct descriptions in [[atomism]] and [[astronomy]], and the [[Ancient Greece|Greek]] mathematician [[Archimedes]] derived many correct quantitative descriptions of [[mechanics]] and [[hydrostatics]]. |
|||
===Middle Ages=== |
|||
{{main|Islamic science|History of science in the Middle Ages}} |
|||
{{see|Science and technology in ancient India|History of science and technology in China}} |
|||
[[Image:Ibn_haithem_portrait.jpg|thumb|right|200px|[[Ibn al-Haitham]] (Alhazen)]] |
|||
The willingness to question previously held truths and search for new answers eventually resulted in a period of major scientific advancements, now known as the [[Scientific Revolution]] of the late [[seventeenth century]]. The precursors to the scientific revolution may be traced back to the important developments made in [[Science and technology in ancient India|Indian science]] and especially [[Islamic science|Muslim science]]. Examples of these developments include including the [[ellipse|elliptical]] model of the planets perhaps based on a [[heliocentrism|heliocentric]] [[solar system]] of [[gravitation]] developed by [[Indian mathematics|Indian mathematician]]-astronomer [[Aryabhata]]; the basic ideas of [[atomic theory]] developed by [[Hindu]] and [[Jaina]] philosophers; the theory of light being equivalent to energy particles developed by the Indian [[Buddhist]] scholars [[Dignāga]] and [[Dharmakirti]]; the [[optics|optical]] theory of [[light]] developed by the [[Iraq]]i scientist [[Ibn al-Haytham]] (Alhazen); the [[Astrolabe]] invented by the [[Persian people|Persian]] [[Islamic astronomy|astronomer]] [[Muhammad al-Fazari]]; and the significant flaws in the [[Ptolemaic system]] pointed out by Persian scientist [[Nasir al-Din Tusi]]. |
|||
The most important scientific development during the [[Middle Ages]], however, was the development of the [[scientific method]], which began with the [[Iraq]]i [[Islamic science|Muslim scientist]] [[Ibn al-Haytham]] ([[Latin]]ized as ''Alhazen''), who pioneered the used of [[experiment]]ation during his investigations on [[optics]] in his ''[[Book of Optics]]''.<ref>Rosanna Gorini (2003). "Al-Haytham the Man of Experience. First Steps in the Science of Vision", ''International Society for the History of Islamic Medicine''. Institute of Neurosciences, Laboratory of Psychobiology and Psychopharmacology, Rome, Italy: |
|||
{{quote|"According to the majority of the historians al-Haytham was the pioneer of the modern scientific method. With his book he changed the meaning of the term optics and established experiments as the norm of proof in the field. His investigations are based not on abstract theories, but on experimental evidences and his experiments were systematic and repeatable."}}</ref> |
|||
===Scientific Revolution=== |
|||
{{main|Scientific Revolution}} |
|||
As the influence of the [[Arab Empire]] expanded to Europe, the works of Aristotle, preserved by the [[Arab]]s, and the works of the Indians and Persians, became known in medieval Europe by the [[twelfth century|twelfth]] and [[thirteenth century|thirteenth centuries]] through [[Latin translations of the 12th century|Latin translations]] of [[Islamic science|Arabic scientific texts]]. |
|||
[[Image:Mikolaj Kopernik.jpg|thumb|150px|left|[[Nicolaus Copernicus]] <!-- do not enter nationality claims here--> 1473-1543]] |
|||
This eventually led to the '''''scientific revolution''''', held by most historians (e.g., Howard Margolis) to have begun in [[1543]], when the first printed copy of [[Nicolaus Copernicus]]'s ''[[De Revolutionibus Orbium Coelestium|De Revolutionibus]]'' was brought to the influential <!-- do not enter nationality claims here--> astronomer from [[Nuremberg]] (Nürnberg), where it had been printed by [[Johannes Petreius]]. Most of its contents had been written years prior, but the publication had been delayed. Copernicus died soon after receiving the copy. |
|||
[[Image:Galileo.arp.300pix.jpg|thumb|150px|right|[[Galileo Galilei]]]] Further significant advances were made over the following century by [[Galileo Galilei]], [[Christiaan Huygens]], [[Johannes Kepler]], and [[Blaise Pascal]]. During the early [[seventeenth century]], Galileo made extensive use of experimentation to validate physical theories, which is the key idea in the modern [[scientific method]]. Galileo formulated and successfully tested several results in [[dynamics (mechanics)|dynamics]], in particular the Law of [[Inertia]]. |
|||
[[Image:GodfreyKneller-IsaacNewton-1689.jpg|thumb|150px|left|[[Sir Isaac Newton]]]] The scientific revolution is considered to have culminated with the publication of the ''[[Philosophiae Naturalis Principia Mathematica]]'' in [[1687]] by the mathematician, physicist, alchemist and inventor Sir [[Isaac Newton]] ([[1643]]-[[1727]]).In [[1687]], [[Isaac Newton|Newton]] published the ''[[Philosophiae Naturalis Principia Mathematica|Principia]]'', detailing two comprehensive and successful physical theories: [[Newton's laws of motion]], from which arise [[classical mechanics]]; and [[gravity|Newton's Law of Gravitation]], which describes the [[fundamental force]] of [[gravity]]. Both theories agreed well with experiment. The Principia also included several theories in [[fluid dynamics]]. |
|||
From the late [[seventeenth century]] onward, [[thermodynamics]] was developed by physicist and chemist [[Robert Boyle|Boyle]], [[Thomas Young (scientist)|Young]], and many others. In [[1733]], [[Daniel Bernoulli|Bernoulli]] used statistical arguments with classical mechanics to derive thermodynamic results, initiating the field of [[statistical mechanics]]. In [[1798]], [[Benjamin Thompson|Thompson]] demonstrated the conversion of mechanical work into heat, and in [[1847]] [[James Joule|Joule]] stated the law of conservation of [[energy]], in the form of heat as well as mechanical energy. [[Ludwig Boltzmann]], in the nineteenth century, is responsible for the modern form of statistical mechanics. |
|||
Classical mechanics was re-formulated and extended by [[Leonhard Euler]], French mathematician [[Joseph Louis Lagrange|Joseph-Louis Comte de Lagrange]], Irish mathematical physicist [[William Rowan Hamilton]], and others, who produced new results in mathematical physics. The law of universal gravitation initiated the field of [[astrophysics]], which describes [[astronomy|astronomical]] phenomena using physical theories. |
|||
After Newton defined [[classical mechanics]], the next great field of inquiry within physics was the nature of [[electricity]]. Observations in the [[seventeenth century|seventeenth]] and [[eighteenth century]] by scientists such as [[Robert Boyle]], [[Stephen Gray (scientist)|Stephen Gray]], and [[Benjamin Franklin]] created a foundation for later work. These observations also established our basic understanding of electrical charge and [[electric current|current]]. |
|||
The existence of the atom was proposed in [[1808]] by [[John Dalton]]. |
|||
<!-- Unsourced image removed: [[Image:James Clerk Maxwell.png|thumb|right|150px|[[James Clerk Maxwell]]]] --> |
|||
In [[1821]], the English physicist and chemist [[Michael Faraday]] integrated the study of [[magnetism]] with the study of electricity. This was done by demonstrating that a moving [[magnet]] induced an [[electric current]] in a [[Electrical conductor|conductor]]. Faraday also formulated a physical conception of [[electromagnetic field]]s. [[James Clerk Maxwell]] built upon this conception, in [[1864]], with an interlinked set of twenty equations that explained the interactions between [[electric field|electric]] and [[magnetic field]]s. These twenty equations were later reduced, using [[vector calculus]], to a set of [[Maxwell's equations|four equations]] by [[Oliver Heaviside]]. |
|||
In addition to other electromagnetic phenomena, Maxwell's equations also can be used to describe [[light]]. Confirmation of this observation was made with the [[1888]] discovery of [[radio]] by [[Heinrich Hertz]] and in [[1895]] when [[Wilhelm Roentgen]] detected [[X-ray]]s. |
|||
===Modern physics=== |
|||
[[Image:Einstein patentoffice.jpg|thumb|left|150px|[[Albert Einstein]]]] |
|||
The ability to describe light in electromagnetic terms helped serve as a springboard for [[Albert Einstein]]'s publication of the theory of [[special relativity]] in 1905. This theory combined classical mechanics with Maxwell's equations. |
|||
The theory of [[special relativity]] unifies space and time into a single entity, [[spacetime]]. Relativity prescribes a different transformation between [[inertial frame of reference|reference frames]] than classical mechanics; this necessitated the development of relativistic mechanics as a replacement for classical mechanics. In the regime of low (relative) velocities, the two theories agree. Einstein built further on the special theory by including gravity into his calculations, and published his theory of [[general relativity]] in [[1915]]. |
|||
One part of the theory of general relativity is [[Einstein's field equation]]. This describes how the ''stress-energy tensor'' creates curvature of [[spacetime]] and forms the basis of general relativity. Further work on Einstein's field equation produced results which predicted the [[Big Bang]], [[black hole]]s, and the [[expanding universe]]. Einstein believed in a static universe. He tried, and failed, to fix his equation to allow for this. By [[1929]], however, [[Edwin Hubble]]'s astronomical observations suggested that the universe is expanding at a possibly exponential rate. |
|||
[[Image:Marie Curie (Nobel-physics).png|thumb|left|150px|[[Marie Sklodowska-Curie]]]] |
|||
In [[1895]], [[Wilhelm Röntgen|Röntgen]] discovered [[X-ray]]s, which turned out to be high-frequency electromagnetic radiation. |
|||
[[Radioactivity]] was discovered in [[1896]] by [[Henri Becquerel]], and further studied by [[Maria Sklodowska-Curie]], [[Pierre Curie]], and others. This initiated the field of [[nuclear physics]]. |
|||
In [[1897]], [[J.J. Thomson|Joseph J. Thomson]] discovered the [[electron]], the elementary particle which carries electrical current in [[electrical circuit|circuits]]. In [[1904]], he proposed the first model of the [[atom]], known as the [[atom/plum pudding|plum pudding model]]. Its existence had been proposed in [[1808]] by [[John Dalton]]. |
|||
These discoveries revealed that the assumption of many physicists, that atoms were the basic unit of [[matter]], was flawed, and prompted further study into the structure of [[atom]]s. |
|||
[[Image:Ernest Rutherford.jpg|thumb|right|150px|[[Ernest Rutherford]]]] |
|||
In [[1911]], [[Ernest Rutherford]] deduced from [[rutherford scattering|scattering experiments]] the existence of a compact atomic nucleus, with positively charged constituents dubbed [[proton]]s. [[Neutron]]s, the neutral nuclear constituents, were discovered in [[1932]] by [[James Chadwick|Chadwick]]. The equivalence of mass and energy (Einstein, 1905) was spectacularly demonstrated during [[World War II]], as research was conducted by each side into [[nuclear physics]], for the purpose of creating a [[nuclear weapon|nuclear bomb]]. The German effort, led by Heisenberg, did not succeed, but the Allied [[Manhattan Project]] reached its goal. In America, a team led by [[Enrico Fermi|Fermi]] achieved the first man-made [[nuclear chain reaction]] in [[1942]], and in [[1945]] the world's first [[nuclear weapon|nuclear explosive]] was detonated at [[Trinity site]], near [[Alamogordo]], [[New Mexico]]. |
|||
In [[1900]], [[Max Planck]] published his explanation of [[blackbody radiation]]. This equation assumed that radiators are [[quantum|quantized]], which proved to be the opening argument in the edifice that would become [[quantum mechanics]]. By introducing discrete energy levels, Planck, Einstein, [[Niels Bohr]], and others developed [[quantum]] theories to explain various anomalous experimental results. |
|||
[[Image:Erwin Schrödinger.jpg|thumb|150px|left| [[Erwin Schrödinger]]]] |
|||
Quantum mechanics was formulated in [[1925]] by [[Werner Heisenberg|Heisenberg]] and in [[1926]] by [[Erwin Schrödinger|Schrödinger]] and [[Paul Dirac]], in two different ways, that both explained the preceding heuristic quantum theories. In quantum mechanics, the outcomes of physical measurements are inherently [[probability|probabilistic]]; the theory describes the calculation of these probabilities. It successfully describes the behavior of matter at small distance scales. During the [[1920s]] Schrödinger, Heisenberg, and [[Max Born]] were able to formulate a consistent picture of the chemical behavior of matter, a complete theory of the electronic structure of the atom, as a byproduct of the quantum theory. |
|||
<!-- Image with unknown copyright status removed: [[Image:Feynman-bongos.jpg|thumb|right|150px|[[Richard Feynman]]]] --> |
|||
[[Quantum field theory]] was formulated in order to extend quantum mechanics to be consistent with special relativity. It was devised in the late [[1940s]] with work by [[Richard Feynman]], [[Julian Schwinger]], [[Sin-Itiro Tomonaga]], and [[Freeman Dyson]]. They formulated the theory of [[quantum electrodynamics]], which describes the electromagnetic interaction, and successfully explained the [[Lamb shift]]. Quantum field theory provided the framework for modern [[particle physics]], which studies [[fundamental force]]s and elementary particles. |
|||
[[Chen Ning Yang]] and [[Tsung-Dao Lee]], in the [[1950s]], discovered an unexpected [[asymmetry]] in the decay of a [[subatomic particle]]. In [[1954]], Yang and [[Robert Mills (physicist)|Robert Mills]] then developed a class of [[gauge theory|gauge theories]] which provided the framework for understanding the nuclear forces (Yang, Mills 1954). The theory for the [[strong nuclear force]] was first proposed by [[Murray Gell-Mann]]. The [[electroweak force]], the unification of the [[weak nuclear force]] with electromagnetism, was proposed by [[Sheldon Lee Glashow]], [[Abdus Salam]], and [[Steven Weinberg]] and confirmed in [[1964]] by [[James Watson Cronin]] and [[Val Fitch]]. This led to the so-called [[Standard Model]] of particle physics in the [[1970s]], which successfully describes all the elementary particles observed to date. |
|||
Quantum mechanics also provided the theoretical tools for [[condensed matter physics]], whose largest branch is [[solid state physics]]. It studies the physical behavior of solids and liquids, including phenomena such as [[crystal structure]]s, [[semiconductor|semiconductivity]], and [[superconductor|superconductivity]]. The pioneers of condensed matter physics include [[Felix Bloch]], who created a quantum mechanical description of the behavior of electrons in crystal structures in [[1928]]. The transistor was developed by physicists [[John Bardeen]], [[Walter Houser Brattain]], and [[William Bradford Shockley]] in [[1947]] at [[Bell Labs|Bell Laboratories]]. |
|||
The two themes of the [[twentieth century]], general relativity and quantum mechanics, appear inconsistent with each other. General relativity describes the [[universe]] on the scale of [[planet]]s and [[solar system]]s, while quantum mechanics operates on sub-atomic scales. This challenge is being attacked by [[string theory]], which treats [[spacetime]] as composed, not of points, but of one-dimensional objects, [[string theory|strings]]. Strings have properties similar to a common string (e.g., [[Tension (mechanics)|tension]] and [[oscillation|vibration]]). The theories yield promising, but not yet testable, results. The search for experimental verification of string theory is in progress. |
|||
[[Image:WYP2005 logo.gif|right|130px]] |
|||
The [[United Nations]] declared the year [[2005]], the centenary of Einstein's [[Annus Mirabilis Papers|annus mirabilis]], as the [[World Year of Physics]]. |
|||
=== Future directions === |
|||
{{main | Unsolved problems in physics}} |
|||
Research in physics is progressing constantly on a large number of fronts, and is likely to do so for the foreseeable future. |
|||
In condensed matter physics, the greatest unsolved theoretical problem is the explanation for [[high-temperature superconductivity]]. Strong efforts, largely experimental, are being put into making workable [[spintronics]] and [[quantum computer]]s. |
|||
In particle physics, the first pieces of experimental evidence for physics beyond the [[Standard Model]] have begun to appear. Foremost amongst these are indications that [[neutrino]]s have non-zero [[mass]]. These experimental results appear to have solved the long-standing [[solar neutrino problem]] in solar physics. The physics of massive neutrinos is currently an area of active theoretical and experimental research. In the next several years, [[particle accelerator]]s will begin probing energy scales in the [[TeV]] range, in which experimentalists are hoping to find evidence for the [[Higgs boson]] and [[supersymmetry|supersymmetric particles]]. |
|||
[[Image:First Gold Beam-Beam Collision Events at RHIC at 100 100 GeV c per beam recorded by STAR.jpg|thumb|right|300px|Thousands of particles explode from the collision point of two relativistic (100 [[GeV]] per ion) [[gold]] ions in the [[STAR detector]] of the [[Relativistic Heavy Ion Collider]]; an experiment done in order to investigate the properties of a [[quark gluon plasma]] such as the one thought to exist in the ultrahot first few microseconds after the [[big bang]]]] |
|||
Theoretical attempts to unify [[quantum mechanics]] and [[general relativity]] into a single theory of [[quantum gravity]], a program ongoing for over half a century, have not yet borne fruit. Currently, the leading candidates are [[M-theory]], [[superstring theory]], and [[loop quantum gravity]]. |
|||
Many [[astronomy|astronomical]] and [[physical cosmology|cosmological]] phenomena have yet to be explained satisfactorily, including the existence of [[GZK paradox|ultra-high energy cosmic rays]], the [[baryon asymmetry]], the [[accelerating universe|acceleration of the universe]], and the [[galaxy rotation problem|anomalous rotation rates of galaxies]]. |
|||
Although much progress has been made in high-energy, [[quantum]], and astronomical physics, many everyday phenomena, involving [[complex systems|complexity]], [[chaos]], or [[turbulence]] remain poorly understood. Complex problems that would appear to be soluble by a clever application of dynamics and mechanics, such as the formation of sand piles, nodes in trickling [[water]], the shape of water [[droplet]]s, mechanisms of [[surface tension]] [[catastrophe theory|catastrophes]], or self-sorting in shaken heterogeneous collections are unsolved. |
|||
These complex phenomena have received growing attention since the 1970s for several reasons, not least of which has been the availability of modern [[mathematics|mathematical]] methods and [[computers]], which enabled [[complex systems]] to be modeled in new ways. The [[interdisciplinary]] [[relevance]] of complex physics also has increased, as exemplified by the study of [[turbulence]] in [[aerodynamics]], or the [[observation]] of [[pattern]] [[formation]] in [[biology|biological]] systems. In 1932, [[Horace Lamb]] correctly prophesied the success of the theory of quantum electrodynamics and the near-stagnant progress in the study of turbulence: |
|||
<blockquote>''I am an old man now, and when I die and go to heaven there are two matters on which I hope for enlightenment. One is quantum electrodynamics, and the other is the turbulent motion of fluids. And about the former I am rather optimistic.''</blockquote> |
|||
== Notes == |
== Notes == |
Revision as of 23:22, 21 July 2007
- This is a discussion of a present category of science. For the work by Aristoteles, see “Physics (Aristotle)”.
Physics (Greek: φύσις (phúsis), "nature" and φυσικῆ (phusiké), "knowledge of nature") is the branch of science concerned with discovering and characterizing universal laws that govern such things as matter, energy, space, and time. Discoveries in physics resonate throughout the natural sciences, and physics has been described as the "fundamental science" because other fields such as chemistry and biology investigate systems whose properties depend on the laws of physics.[1]
The emergence of physics as a science distinct from natural philosophy began with the scientific revolution of the 16th and 17th centuries and continued through the dawn of modern physics in the early 20th century. The field has continued to expand, with a growing body of research leading to discoveries such as the Standard Model of fundamental particles and a detailed history of the universe, along with revolutionary new technologies like nuclear weapons and semiconductors. Research today progresses on a vast array of topics, including high-temperature superconductivity, quantum computing, the search for the Higgs boson, and the attempt to develop a theory of quantum gravity. Grounded in observations and experiments and supported by deep, far-reaching theories, physics has made a multitude of contributions to science, technology, and philosophy.
Experimental physics is closely related to engineering and technology. Physicists involved in basic research design and perform experiments with equipment such as particle accelerators and lasers, whereas physicists involved in applied research invent technologies such as magnetic resonance imaging (MRI) and transistors.
Theoretical physics is closely related to mathematics, which provides the language of physical theories. Theoretical physicists may also rely on numerical analysis and computer simulations, which play an ever richer role in the formulation of physical models. The fields of mathematical and computational physics are active areas of research. Theoretical physics sometimes relates to philosophy and metaphysics when it deals with speculative ideas like multidimensional spaces and parallel universes.
Core Theories
Although physics encompasses a wide variety of phenomena, there are basic theories that are known to all physicists. These theories have been tested in numerous experiments and proven to be correct approximations of nature within their domains of validity. For example, classical mechanics accurately describes the motion of objects in everyday experience, but it breaks down at the atomic scale and at speeds approaching the speed of light. The basic theories are important tools for research into more specialized topics. A table of the basic theories of physics, along with many of the concepts they employ, can be found here.
Classical Mechanics

Classical mechanics is a model of the physics of forces acting upon bodies. It is often referred to as "Newtonian mechanics" after Newton and his laws of motion. Classical mechanics is subdivided into statics, which models objects at rest, kinematics, which models objects in motion, and dynamics, which models objects subjected to forces. It is superseded by relativistic mechanics for systems moving at large velocities near the speed of light, quantum mechanics for systems at small distance scales, and relativistic quantum field theory for systems with both properties. Nevertheless, classical mechanics is still very useful, because it is much simpler and easier to apply than these other theories, and it has a very large range of approximate validity. Classical mechanics can be used to describe the motion of human-sized objects (such as tops and baseballs), many astronomical objects (such as planets and galaxies), and certain microscopic objects (such as organic molecules.)
Electromagnetism
Electromagnetism (EM) is a branch of physics in which electric and magnetic phenomena are studied -- called also Electromagnetics. Electromagnetism may be regarded as the study of the interactions between electric charges at rest (Electrostatics) and in motion (Electrodynamics). It entails the analysis, synthesis, physical interpretation, and application of electric and magnetic fields. The subject of electromagnetic phenomena can be summarized in Maxwell's equations; Maxwell based these equations on previously known results, both experimental and theoretical.
Electrostatics is the study of phenomena associated with charged bodies at rest. Such bodies exert forces on each other, as described by Coulomb’s law, and their behavior can be analyzed in terms of the concept of an electric field surrounding any charged body such that another charged body located at any point in the field is subject to a force proportional to the magnitude of its charge and its attraction or repulsion, depending on the polarity of the charge. Electrostatics has many applications, ranging from the analysis of phenomena such as thunderstorms to the study of the behavior of electron tubes.
Electrodynamics is the study of phenomena associated with charged bodies in motion and varying electric and magnetic fields (see charge; electricity); since a moving charge produces a magnetic field, electrodynamics is concerned with effects such as magnetism, electromagnetic radiation, and electromagnetic induction, including such practical applications as the electric generator and the electric motor. This area of electrodynamics, often known as classical electrodynamics, was first systematically explained by the physicist James Clerk Maxwell. Maxwell’s equations, a set of differential equations, describe the phenomena of this area with great generality. A more recent development is quantum electrodynamics, which was formulated to explain the interaction of electromagnetic radiation with matter, to which the laws of the quantum theory apply. The physicists P. A. M. Dirac, W. Heisenberg, and W. Pauli were the pioneers in the formulation of quantum electrodynamics. When the velocities of the charged particles under consideration become comparable with the speed of light, corrections involving the theory of relativity must be made; this branch of the theory is called relativistic electrodynamics. It is applied to phenomena involved with particle accelerators and with electron tubes that are subject to high voltages and carry heavy currents.
Electromagnetism encompasses various real-world electromagnetic phenomena. In fact, light is an oscillating electromagnetic field that is radiated from accelerating charged particles. Aside from gravity, most of the forces in everyday experience are ultimately a result of electromagnetism.
EM principles find applications in various allied disciplines such as microwaves, antennas, electric machines, satellite communications, bioelectromagnetics, plasmas, nuclear research, fiber optics, electromagnetic interference and compatibility, electromechanical energy conversion, radar meteorology, and remote sensing. EM devices include transformers, electric relays, radio/TV, telephones, electric motors, transmission lines, waveguides, antennas, optical fibers, radars, and lasers. The design of these devices requires thorough knowledge of the laws and principles of EM.
Thermodynamics

Thermodynamics is the branch of physics that deals with the action of heat and the conversions from one to another of various forms of energy. Thermodynamics is particularly concerned with how these affect temperature, pressure, volume, mechanical action, entropy, and work.
Statistical Mechanics
Statistical mechanics is the branch of physics that analyzes macroscopic systems by applying statistical principles to their microscopic constituents. It can be applied to calculate the thermodynamic properties of bulk materials from the properties of individual molecules, which is the basis of statistical thermodynamics.
Quantum mechanics

Quantum mechanics is the branch of mathematical physics treating atomic and subatomic systems and their interaction with radiation in terms of observable quantities. It is based on the observation that all forms of energy are released in discrete units or bundles called quanta. Quantum mechanics provides a physical theory of matter that is based on the concept of the possession of wave properties by elementary particles, affords a mathematical interpretation of the structure and interactions of matter on the basis of these properties, and incorporates within it quantum theory and the uncertainty principle -- called also wave mechanics. Remarkably, quantum theory typically permits only probable or statistical calculation of the observed features of subatomic particles, understood in terms of wavefunctions. The discovery of quantum mechanics in the early 20th century revolutionized physics, and quantum mechanics is fundamental to most areas of current research.
Relativity
Relativity is a generalization of classical mechanics that describes fast-moving or very massive systems. It includes special and general relativity.
Special relativity, or the "special theory of relativity", is based on two postulates:
- The mathematical forms of the laws of physics are invariant in all inertial systems.
- The speed of light in a vacuum is constant and independent of the source or observer.
In special relativity, there is an equivalence of mass and energy and a change in length and time with increased velocity.
General relativity, or the "general theory of relativity", extends special relativity to include transformations between non-inertial frames. It is formulated using differential geometry and interprets gravity as a distortion of spacetime caused by the presence of mass or energy.
Research
Contemporary research in physics can be broadly divided into condensed matter physics; atomic, molecular, and optical physics; particle physics; and astrophysics. Since the twentieth century, the individual fields of physics have become increasingly specialized, and today most physicists work in a single field for their entire careers. "Universalists" such as Albert Einstein (1879–1955) and Lev Landau (1908–1968), who worked in multiple fields of physics, are now very rare. A table of the major fields of physics, along with their subfields and the theories they employ can be found here.
Theory and experiment
The culture of physics research differs from most sciences in the separation of theory and experiment. Since the twentieth century, most individual physicists have specialized in either theoretical physics or experimental physics. The great Italian physicist Enrico Fermi (1901–1954), who made fundamental contributions to both theory and experimentation in nuclear physics, was a notable exception. In contrast, almost all the successful theorists in biology and chemistry (e.g. American quantum chemist and biochemist Linus Pauling) have also been experimentalists, although this is changing as of late.
Roughly speaking, theorists seek to develop through abstractions and mathematical models theories that can both describe and interpret existing experimental results, and successfully predict future results, while experimentalists devise and perform experiments to explore new phenomena and test theoretical predictions. Although theory and experiment are developed separately, they are strongly dependent upon each other. Progress in physics frequently comes about when experimentalists make a discovery that existing theories cannot account for, necessitating the formulation of new theories. Likewise, ideas arising from theory often inspire new experiments. In the absence of experiment, theoretical research can go in the wrong direction; this is one of the criticisms that has been leveled against M-theory, a popular theory in high-energy physics for which no practical experimental test has ever been devised. Theorists working closely with experimentalists frequently employ phenomenology.
High energy
Particle physics, also known as "high-energy physics", is concerned with the properties of submicroscopic particles much smaller than atoms, including elementary particles such as electrons, photons, and quarks. A topic of current interest is the search for the Higgs boson.
Condensed matter
Condensed matter physics is concerned with how the properties of bulk matter, such as the ordinary solids and liquids we encounter in everyday life, arise from the properties and mutual interactions of the constituent atoms. A topic of current interest is high-temperature superconductivity.
AMO
Atomic, molecular, and optical physics (AMO) deals with small numbers of atoms and molecules, particularly with how they interact with light. A topic of current interest is the behavior of Bose-Einstein condensates.
Astrophysics

Astrophysics and cosmology apply the laws of physics to explain celestial phenomena, including stellar dynamics, black holes, galaxies, and the big bang. A topic of current interest is determining the nature of dark matter and dark energy.
Applied physics
Applied physics is physics that is intended for a particular technological or practical use, as for example in engineering, as opposed to basic research. Applied physics is rooted in the fundamental truths and basic concepts of the physical sciences, but is concerned with the use of scientific principles in practical devices and systems, and in the application of physics in other areas of science. The approach is similar to that of applied mathematics. "Applied" is distinguished from "pure" by a subtle combination of factors such as the motivation and attitude of researchers and the nature of the relationship to the technology or science that may be affected by the work. [1]
History
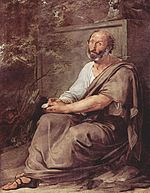
Since antiquity, people have tried to understand the workings of Nature and the behavior of matter: why unsupported objects drop to the ground, why different materials have different properties, and so forth. The character of the universe was also a mystery, for instance the earth and the behavior of celestial objects such as the sun and the moon. Several theories were proposed, most of which were incorrect, such as the earth orbiting the moon. These first theories were largely couched in philosophical terms, and never verified by systematic experimental testing, as is popular today. The works of Ptolemy and Aristotle were not always found to match everyday observations. There were exceptions and there are anachronisms - for example, Indian philosophers and astronomers gave many correct descriptions in atomism and astronomy, and the Greek mathematician Archimedes derived many correct quantitative descriptions of mechanics and hydrostatics.
Middle Ages
The willingness to question previously held truths and search for new answers eventually resulted in a period of major scientific advancements, now known as the Scientific Revolution of the late seventeenth century. The precursors to the scientific revolution may be traced back to the important developments made in Indian science and especially Muslim science. Examples of these developments include including the elliptical model of the planets perhaps based on a heliocentric solar system of gravitation developed by Indian mathematician-astronomer Aryabhata; the basic ideas of atomic theory developed by Hindu and Jaina philosophers; the theory of light being equivalent to energy particles developed by the Indian Buddhist scholars Dignāga and Dharmakirti; the optical theory of light developed by the Iraqi scientist Ibn al-Haytham (Alhazen); the Astrolabe invented by the Persian astronomer Muhammad al-Fazari; and the significant flaws in the Ptolemaic system pointed out by Persian scientist Nasir al-Din Tusi.
The most important scientific development during the Middle Ages, however, was the development of the scientific method, which began with the Iraqi Muslim scientist Ibn al-Haytham (Latinized as Alhazen), who pioneered the used of experimentation during his investigations on optics in his Book of Optics.[2]
Scientific Revolution
As the influence of the Arab Empire expanded to Europe, the works of Aristotle, preserved by the Arabs, and the works of the Indians and Persians, became known in medieval Europe by the twelfth and thirteenth centuries through Latin translations of Arabic scientific texts.

This eventually led to the scientific revolution, held by most historians (e.g., Howard Margolis) to have begun in 1543, when the first printed copy of Nicolaus Copernicus's De Revolutionibus was brought to the influential astronomer from Nuremberg (Nürnberg), where it had been printed by Johannes Petreius. Most of its contents had been written years prior, but the publication had been delayed. Copernicus died soon after receiving the copy.

Further significant advances were made over the following century by Galileo Galilei, Christiaan Huygens, Johannes Kepler, and Blaise Pascal. During the early seventeenth century, Galileo made extensive use of experimentation to validate physical theories, which is the key idea in the modern scientific method. Galileo formulated and successfully tested several results in dynamics, in particular the Law of Inertia.

The scientific revolution is considered to have culminated with the publication of the Philosophiae Naturalis Principia Mathematica in 1687 by the mathematician, physicist, alchemist and inventor Sir Isaac Newton (1643-1727).In 1687, Newton published the Principia, detailing two comprehensive and successful physical theories: Newton's laws of motion, from which arise classical mechanics; and Newton's Law of Gravitation, which describes the fundamental force of gravity. Both theories agreed well with experiment. The Principia also included several theories in fluid dynamics.
From the late seventeenth century onward, thermodynamics was developed by physicist and chemist Boyle, Young, and many others. In 1733, Bernoulli used statistical arguments with classical mechanics to derive thermodynamic results, initiating the field of statistical mechanics. In 1798, Thompson demonstrated the conversion of mechanical work into heat, and in 1847 Joule stated the law of conservation of energy, in the form of heat as well as mechanical energy. Ludwig Boltzmann, in the nineteenth century, is responsible for the modern form of statistical mechanics.
Classical mechanics was re-formulated and extended by Leonhard Euler, French mathematician Joseph-Louis Comte de Lagrange, Irish mathematical physicist William Rowan Hamilton, and others, who produced new results in mathematical physics. The law of universal gravitation initiated the field of astrophysics, which describes astronomical phenomena using physical theories.
After Newton defined classical mechanics, the next great field of inquiry within physics was the nature of electricity. Observations in the seventeenth and eighteenth century by scientists such as Robert Boyle, Stephen Gray, and Benjamin Franklin created a foundation for later work. These observations also established our basic understanding of electrical charge and current.
The existence of the atom was proposed in 1808 by John Dalton.
In 1821, the English physicist and chemist Michael Faraday integrated the study of magnetism with the study of electricity. This was done by demonstrating that a moving magnet induced an electric current in a conductor. Faraday also formulated a physical conception of electromagnetic fields. James Clerk Maxwell built upon this conception, in 1864, with an interlinked set of twenty equations that explained the interactions between electric and magnetic fields. These twenty equations were later reduced, using vector calculus, to a set of four equations by Oliver Heaviside.
In addition to other electromagnetic phenomena, Maxwell's equations also can be used to describe light. Confirmation of this observation was made with the 1888 discovery of radio by Heinrich Hertz and in 1895 when Wilhelm Roentgen detected X-rays.
Modern physics

The ability to describe light in electromagnetic terms helped serve as a springboard for Albert Einstein's publication of the theory of special relativity in 1905. This theory combined classical mechanics with Maxwell's equations. The theory of special relativity unifies space and time into a single entity, spacetime. Relativity prescribes a different transformation between reference frames than classical mechanics; this necessitated the development of relativistic mechanics as a replacement for classical mechanics. In the regime of low (relative) velocities, the two theories agree. Einstein built further on the special theory by including gravity into his calculations, and published his theory of general relativity in 1915.
One part of the theory of general relativity is Einstein's field equation. This describes how the stress-energy tensor creates curvature of spacetime and forms the basis of general relativity. Further work on Einstein's field equation produced results which predicted the Big Bang, black holes, and the expanding universe. Einstein believed in a static universe. He tried, and failed, to fix his equation to allow for this. By 1929, however, Edwin Hubble's astronomical observations suggested that the universe is expanding at a possibly exponential rate.
In 1895, Röntgen discovered X-rays, which turned out to be high-frequency electromagnetic radiation.
Radioactivity was discovered in 1896 by Henri Becquerel, and further studied by Maria Sklodowska-Curie, Pierre Curie, and others. This initiated the field of nuclear physics.
In 1897, Joseph J. Thomson discovered the electron, the elementary particle which carries electrical current in circuits. In 1904, he proposed the first model of the atom, known as the plum pudding model. Its existence had been proposed in 1808 by John Dalton.
These discoveries revealed that the assumption of many physicists, that atoms were the basic unit of matter, was flawed, and prompted further study into the structure of atoms.
In 1911, Ernest Rutherford deduced from scattering experiments the existence of a compact atomic nucleus, with positively charged constituents dubbed protons. Neutrons, the neutral nuclear constituents, were discovered in 1932 by Chadwick. The equivalence of mass and energy (Einstein, 1905) was spectacularly demonstrated during World War II, as research was conducted by each side into nuclear physics, for the purpose of creating a nuclear bomb. The German effort, led by Heisenberg, did not succeed, but the Allied Manhattan Project reached its goal. In America, a team led by Fermi achieved the first man-made nuclear chain reaction in 1942, and in 1945 the world's first nuclear explosive was detonated at Trinity site, near Alamogordo, New Mexico.
In 1900, Max Planck published his explanation of blackbody radiation. This equation assumed that radiators are quantized, which proved to be the opening argument in the edifice that would become quantum mechanics. By introducing discrete energy levels, Planck, Einstein, Niels Bohr, and others developed quantum theories to explain various anomalous experimental results.
Quantum mechanics was formulated in 1925 by Heisenberg and in 1926 by Schrödinger and Paul Dirac, in two different ways, that both explained the preceding heuristic quantum theories. In quantum mechanics, the outcomes of physical measurements are inherently probabilistic; the theory describes the calculation of these probabilities. It successfully describes the behavior of matter at small distance scales. During the 1920s Schrödinger, Heisenberg, and Max Born were able to formulate a consistent picture of the chemical behavior of matter, a complete theory of the electronic structure of the atom, as a byproduct of the quantum theory.
Quantum field theory was formulated in order to extend quantum mechanics to be consistent with special relativity. It was devised in the late 1940s with work by Richard Feynman, Julian Schwinger, Sin-Itiro Tomonaga, and Freeman Dyson. They formulated the theory of quantum electrodynamics, which describes the electromagnetic interaction, and successfully explained the Lamb shift. Quantum field theory provided the framework for modern particle physics, which studies fundamental forces and elementary particles.
Chen Ning Yang and Tsung-Dao Lee, in the 1950s, discovered an unexpected asymmetry in the decay of a subatomic particle. In 1954, Yang and Robert Mills then developed a class of gauge theories which provided the framework for understanding the nuclear forces (Yang, Mills 1954). The theory for the strong nuclear force was first proposed by Murray Gell-Mann. The electroweak force, the unification of the weak nuclear force with electromagnetism, was proposed by Sheldon Lee Glashow, Abdus Salam, and Steven Weinberg and confirmed in 1964 by James Watson Cronin and Val Fitch. This led to the so-called Standard Model of particle physics in the 1970s, which successfully describes all the elementary particles observed to date.
Quantum mechanics also provided the theoretical tools for condensed matter physics, whose largest branch is solid state physics. It studies the physical behavior of solids and liquids, including phenomena such as crystal structures, semiconductivity, and superconductivity. The pioneers of condensed matter physics include Felix Bloch, who created a quantum mechanical description of the behavior of electrons in crystal structures in 1928. The transistor was developed by physicists John Bardeen, Walter Houser Brattain, and William Bradford Shockley in 1947 at Bell Laboratories.
The two themes of the twentieth century, general relativity and quantum mechanics, appear inconsistent with each other. General relativity describes the universe on the scale of planets and solar systems, while quantum mechanics operates on sub-atomic scales. This challenge is being attacked by string theory, which treats spacetime as composed, not of points, but of one-dimensional objects, strings. Strings have properties similar to a common string (e.g., tension and vibration). The theories yield promising, but not yet testable, results. The search for experimental verification of string theory is in progress.
The United Nations declared the year 2005, the centenary of Einstein's annus mirabilis, as the World Year of Physics.
Future directions
Research in physics is progressing constantly on a large number of fronts, and is likely to do so for the foreseeable future.
In condensed matter physics, the greatest unsolved theoretical problem is the explanation for high-temperature superconductivity. Strong efforts, largely experimental, are being put into making workable spintronics and quantum computers.
In particle physics, the first pieces of experimental evidence for physics beyond the Standard Model have begun to appear. Foremost amongst these are indications that neutrinos have non-zero mass. These experimental results appear to have solved the long-standing solar neutrino problem in solar physics. The physics of massive neutrinos is currently an area of active theoretical and experimental research. In the next several years, particle accelerators will begin probing energy scales in the TeV range, in which experimentalists are hoping to find evidence for the Higgs boson and supersymmetric particles.
Theoretical attempts to unify quantum mechanics and general relativity into a single theory of quantum gravity, a program ongoing for over half a century, have not yet borne fruit. Currently, the leading candidates are M-theory, superstring theory, and loop quantum gravity.
Many astronomical and cosmological phenomena have yet to be explained satisfactorily, including the existence of ultra-high energy cosmic rays, the baryon asymmetry, the acceleration of the universe, and the anomalous rotation rates of galaxies.
Although much progress has been made in high-energy, quantum, and astronomical physics, many everyday phenomena, involving complexity, chaos, or turbulence remain poorly understood. Complex problems that would appear to be soluble by a clever application of dynamics and mechanics, such as the formation of sand piles, nodes in trickling water, the shape of water droplets, mechanisms of surface tension catastrophes, or self-sorting in shaken heterogeneous collections are unsolved.
These complex phenomena have received growing attention since the 1970s for several reasons, not least of which has been the availability of modern mathematical methods and computers, which enabled complex systems to be modeled in new ways. The interdisciplinary relevance of complex physics also has increased, as exemplified by the study of turbulence in aerodynamics, or the observation of pattern formation in biological systems. In 1932, Horace Lamb correctly prophesied the success of the theory of quantum electrodynamics and the near-stagnant progress in the study of turbulence:
I am an old man now, and when I die and go to heaven there are two matters on which I hope for enlightenment. One is quantum electrodynamics, and the other is the turbulent motion of fluids. And about the former I am rather optimistic.
Notes
- ^ The Feynman Lectures on Physics Volume I, Chapter III. Feynman, Leighton and Sands. ISBN 0-201-02115-3 For the philosophical issues of whether other sciences can be "reduced" to physics, see reductionism and special sciences.
- ^ Rosanna Gorini (2003). "Al-Haytham the Man of Experience. First Steps in the Science of Vision", International Society for the History of Islamic Medicine. Institute of Neurosciences, Laboratory of Psychobiology and Psychopharmacology, Rome, Italy:
"According to the majority of the historians al-Haytham was the pioneer of the modern scientific method. With his book he changed the meaning of the term optics and established experiments as the norm of proof in the field. His investigations are based not on abstract theories, but on experimental evidences and his experiments were systematic and repeatable."
Further reading
- A large number of textbooks, popular books, and webpages about physics are available for further reading.
- Important publications in physics
Organizations
- AIP.org is the website of the American Institute of Physics
- IOP.org is the website of the Institute of Physics
- APS.org is the website of the American Physical Society
- SPS National is the website of the American Society of Physics Students
- CAP.ca is the website of the Canadian Association of Physicists
- EPS.org is the website of the European Physical Society
References
- Yang, Mills 1954 Physical Review 95, 631; Yang, Mills 1954 Physical Review 96, 191.