Hard disk drive
![]() A hard disk drive with the metal cover removed. | |
Date invented | December 14, 1954 |
---|---|
Invented by | An IBM team led by Rey Johnson |
Connects to | Host adapter of system, in PCs typically integrated into motherboard. via one of: |
Market Segments | Desktop computers Mobile computing Enterprise computing Consumer electronic |
A hard disk drive[1] (often shortened as "hard disk"[2] or "hard drive"[3]), is a non-volatile storage device which stores digitally encoded data on rapidly rotating platters with magnetic surfaces. Strictly speaking, "drive" refers to a device distinct from its medium, such as a tape drive and its tape, or a floppy disk drive and its floppy disk. Early HDDs had removable media; however, an HDD today is typically a sealed unit (except for a filtered vent hole to equalize air pressure) with fixed media.[4][5]
History
HDDs (introduced in 1956 as data storage for an IBM accounting computer[6]) were originally developed for use with general purpose computers. During the 1990s, the need for large-scale, reliable storage, independent of a particular device, led to the introduction of embedded systems such as RAIDs, network attached storage (NAS) systems and storage area network (SAN) systems that provide efficient and reliable access to large volumes of data. In the 21st century, HDD usage expanded into consumer applications such as camcorders, cellphones (e.g. the Nokia N91), digital audio players, digital video players (e.g. the iPod Classic), digital video recorders, personal digital assistants and video game consoles.
Technology

HDDs record data by magnetizing ferromagnetic material directionally, to represent either a 0 or a 1 binary digit. They read the data back by detecting the magnetization of the material. A typical HDD design consists of a spindle which holds one or more flat circular disks called platters, onto which the data are recorded. The platters are made from a non-magnetic material, usually aluminum alloy or glass, and are coated with a thin layer of magnetic material. Older disks used iron(III) oxide as the magnetic material, but current disks use a cobalt-based alloy. [citation needed]

The platters are spun at very high speeds. Information is written to a platter as it rotates past devices called read-and-write heads that operate very close (tens of nanometers in new drives) over the magnetic surface. The read-and-write head is used to detect and modify the magnetization of the material immediately under it. There is one head for each magnetic platter surface on the spindle, mounted on a common arm. An actuator arm (or access arm) moves the heads on an arc (roughly radially) across the platters as they spin, allowing each head to access almost the entire surface of the platter as it spins. The arm is moved using a voice coil actuator or in some older designs a stepper motor.
The magnetic recording media are CoCrPt-based magnetic thin films of about 10-20 nm in thickness. The thin films are normally deposited on glass/ceramic/metal substrate and covered by thin carbon layer for protection. The Co-based alloy thin films are polycrystalline and the size of grains has an order of 10 nm. Because the sizes of each grain are tiny, they are typical single domain magnets. The media are magnetically hard (coercivity is about 0.3T) so that a stable remnant magnetization can be achieved. The grain boundaries turn out to be very important. The reason is that, the grains are very small and close to each other, so the coupling between each grains are very strong. When one grain is magnetized, the adjacent grains tend to be aligned parallel to it or demagnetized. Then both the stability of the data and signal-to-noise ratio will be sabotaged. A clear grain boundary can weaken the coupling of the grains and subsequently increase the signal-to-noise ratio. During writing process, ideally one grain can store one bit (1/0). However, current technology can not reach that far yet. In practice, a group of grains (about 100) are magnetized as one bit. So, in order to increase the data density, smaller grains are required. From microstructure point of view, longitudinal and perpendicular recording are the same. Also, similar Co-based thin films are used in both longitudinal and perpendicular recording. However, the fabrication processes are different to gain different crystal structure and magnetic properties. In longitudinal recording, the single-domain grains have uniaxial anisotropy with easy axes lying in the film plane. The consequence of this arrangement is that adjacent magnets repel each other. Therefore the magnetostatic energy is so large that it is difficult to increase areal density. Perpendicular recording media, on the other hand, has the easy axis of the grains oriented perpendicular to the disk plane. Adjacent magnets attract to each other and magnetostatic energy are much lower. So, much higher areal density can be achieved in perpendicular recording. Another unique feature in perpendicular recording is that a soft magnetic underlayer are incorporated into the recording disk.This underlayer is used to conduct writing magnetic flux so that the writing is more efficient. This will be discussed in writing process. Therefore, a higher anisotropy medium film, such as L10-FePt and rare-earth magnets, can be used.
Older drives read the data on the platter by sensing the rate of change of the magnetism in the head; these heads had small coils, and worked (in principle) much like magnetic-tape playback heads, although not in contact with the recording surface. As data density increased, read heads using magnetoresistance (MR) came into use; the electrical resistance of the head changed according to the strength of the magnetism from the platter. Later development made use of spintronics; in these heads, the magnetoresistive effect was much greater than in earlier types, and was dubbed "giant" magnetoresistance (GMR). This refers to the degree of effect, not the physical size, of the head — the heads themselves are extremely tiny, and are too small to be seen without a microscope. GMR read heads are now commonplace.[citation needed]
HD heads are kept from contacting the platter surface by the air that is extremely close to the platter; that air moves at, or close to, the platter speed.[citation needed] The record and playback head are mounted on a block called a slider, and the surface next to the platter is shaped to keep it just barely out of contact. It's a type of air bearing.
The magnetic surface of each platter is conceptually divided into many small sub-micrometre-sized magnetic regions, each of which is used to encode a single binary unit of information. In today's HDDs, each of these magnetic regions is composed of a few hundred magnetic grains. Each magnetic region forms a magnetic dipole which generates a highly localized magnetic field nearby. The write head magnetizes a region by generating a strong local magnetic field. Early HDDs used an electromagnet both to generate this field and to read the data by using electromagnetic induction. Later versions of inductive heads included metal in Gap (MIG) heads and thin film heads. In today's heads, the read and write elements are separate, but in close proximity, on the head portion of an actuator arm. The read element is typically magneto-resistive while the write element is typically thin-film inductive.[7]
In modern drives, the small size of the magnetic regions creates the danger that their magnetic state might be lost because of thermal effects. To counter this, the platters are coated with two parallel magnetic layers, separated by a 3-atom-thick layer of the non-magnetic element ruthenium, and the two layers are magnetized in opposite orientation, thus reinforcing each other.[8] Another technology used to overcome thermal effects to allow greater recording densities is perpendicular recording, first shipped in 2005,[9] as of 2007 the technology was used in many HDDs.[10][11][12]
Error handling
Modern drives also make extensive use of Error Correcting Codes (ECCs), particularly Reed–Solomon error correction. These techniques store extra bits for each block of data that are determined by mathematical formulas. The extra bits allow many errors to be fixed. While these extra bits take up space on the hard drive, they allow higher recording densities to be employed, resulting in much larger storage capacity for user data. [13]
Typical hard drives attempt to "remap" the data in a physical sector that is going bad to a spare physical sector -- hopefully while the number of errors in that bad sector is still small enough that the ECC can completely recover the data without loss. The S.M.A.R.T. system counts the total number of errors in the entire hard drive fixed by ECC, and the total number of remappings, in an attempt to predict hard drive failure.
Architecture

A typical hard drive has two electric motors, one to spin the disks and one to position the read/write head assembly. The disk motor has an external rotor attached to the platters; the stator windings are fixed in place. The actuator has a read-write head under the tip of its very end (near center); a thin printed-circuit cable connects the read-write head to the hub of the actuator. A flexible, somewhat 'U'-shaped, ribbon cable, seen edge-on below and to the left of the actuator arm in the first image and more clearly in the second, continues the connection from the head to the controller board on the opposite side.
The head support arm is very light, but also rigid; in modern drives, acceleration at the head reaches 250 Gs.

The silver-colored structure at the upper left of the first image is the top plate of the permanent-magnet and moving coil motor that swings the heads to the desired position (it is shown removed in the second image). The plate supports a thin neodymium-iron-boron (NIB) high-flux magnet. Beneath this plate is the moving coil, often referred to as the voice coil by analogy to the coil in loudspeakers, which is attached to the actuator hub, and beneath that is a second NIB magnet, mounted on the bottom plate of the motor (some drives only have one magnet).
The voice coil, itself, is shaped rather like an arrowhead, and made of doubly-coated copper magnet wire. The inner layer is insulation, and the outer is thermoplastic, which bonds the coil together after it's wound on a form, making it self-supporting. The portions of the coil along the two sides of the arrowhead (which point to the actuator bearing center) interact with the magnetic field, developing a tangential force that rotates the actuator. Current flowing radially outward along one side of the arrowhead, and radially inward on the other produces the tangential force. (See magnetic field#Force on a charged particle.) If the magnetic field were uniform, each side would generate opposing forces that would cancel each other out. Therefore the surface of the magnet is half N pole, half S pole, with the radial dividing line in the middle, causing the two sides of the coil to see opposite magnetic fields and produce forces that add instead of canceling. Currents along the top and bottom of the coil produce radial forces that do not rotate the head.
Capacity and access speed
![]() | It has been suggested that Disk Overhead and Talk:Disk Overhead#Merge proposal be merged into this article. (Discuss) Proposed since March 2008. |
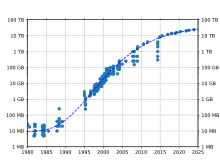
Using rigid disks and sealing the unit allows much tighter tolerances than in a floppy disk drive. Consequently, hard disk drives can store much more data than floppy disk drives and can access and transmit it faster.
- As of April 2009[update], the highest capacity HDDs are 2 TB.[14]
- A typical "desktop HDD" might store between 120 GB and 2 TB although rarely above 500GB of data (based on US market data[15]) rotate at 5,400 to 10,000 rpm and have a media transfer rate of 1 Gbit/s or higher. Some newer have 3Gbit/s.[citation needed] (1 GB = 109 B; 1 Gbit/s = 109 bit/s)
- The fastest “enterprise” HDDs spin at 10,000 or 15,000 rpm, and can achieve sequential media transfer speeds above 1.6 Gbit/s.[16] and a sustained transfer rate up to 125 MBytes/second.[16] Drives running at 10,000 or 15,000 rpm use smaller platters to mitigate increased power requirements (due to air drag) and therefore generally have lower capacity than the highest capacity desktop drives.
- "Mobile HDDs", i.e., laptop HDDs, which are physically smaller than their desktop and enterprise counterparts, tend to be slower and have lower capacity. A typical mobile HDD spins at 5,400 rpm, with 7,200 rpm models available for a slight price premium. Because of the smaller disks, mobile HDDs generally have lower capacity than the highest capacity desktop drives.
The exponential increases in disk space and data access speeds of HDDs have enabled the commercial viability of consumer products that require large storage capacities, such as digital video recorders and digital audio players.[17] In addition, the availability of vast amounts of cheap storage has made viable a variety of web-based services with extraordinary capacity requirements, such as free-of-charge web search, web archiving and video sharing (Google, Internet Archive, YouTube, etc.).
The main way to decrease access time is to increase rotational speed, thus reducing rotational delay, while the main way to increase throughput and storage capacity is to increase areal density. Based on historic trends, analysts predict a future growth in HDD bit density (and therefore capacity) of about 40% per year.[18] Access times have not kept up with throughput increases, which themselves have not kept up with growth in storage capacity.
The first 3.5″ HDD marketed as able to store 1 TB was the Hitachi Deskstar 7K1000. It contains five platters at approximately 200 GB each, providing 935.5 GiB of usable space;[19] note the discrepancy between its capacity in decimal units (1 TB = 1012 bytes) and binary units (1 TiB = 1024 GiB = 240 bytes). Hitachi has since been joined by Samsung (Samsung SpinPoint F1, which has 3 × 334 GB platters), Seagate and Western Digital in the 1 TB drive market.[20][21]
As of December 2008 a single 3.5" platter is able to hold 500GB worth of data.[22]
Form factor | Width | Largest capacity | Platters (Max) |
---|---|---|---|
5.25″ FH | 146 mm | 47 GB[23] (1998) | 14 |
5.25″ HH | 146 mm | 19.3 GB[24] (1998) | 4[25] |
3.5″ | 102 mm | 2 TB[26] (2009) | 5 |
2.5″ | 69.9 mm | 500 GB[27] (2008) | 3 |
1.8″ (CE-ATA/ZIF) | 54 mm | 250 GB[28] (2008) | 3 |
1.3″ | 43 mm | 40 GB[29] (2007) | 1 |
1″ (CFII/ZIF/IDE-Flex) | 42 mm | 20 GB (2006) | 1 |
0.85″ | 24 mm | 8 GB[30] (2004) | 1 |
Capacity measurements

Raw unformatted capacity of a hard disk drive is usually quoted with SI prefixes (metric system prefixes), incrementing by powers of 1000; today that usually means Gigabytes (GB) and Terabytes(TB). This is conventional for data speeds and memory sizes which are not inherently manufactured in power of two sizes, as RAM and Flash memory are. Hard disks by contrast have no inherent binary size as capacity is determined by number of heads, tracks and sectors.
This can cause some confusion because some operating systems may report the formatted capacity of a hard drive using binary prefix units which increment by powers of 1024; today that would be Gibibytes (GiB) and Tebibytes (TiB). To make matters more confusing, these reports may be mislabeled as GB and TB rather than GiB and TiB.
A one Terabyte (1 TB) disk drive would be expected to hold around 1 trillion bytes (1,000,000,000,000) or 1000 GB; and indeed most 1 TB hard drives will contain slightly more than this number. However some Operating System utilities would report this correctly as around 931 GiB or 953,674 MiB, although the report might be incorrectly displayed as 931 GB or 953,674 MB, using the wrong labels. (The actual number for a formatted capacity will be somewhat smaller still, depending on the file system). The following are all correct, if possibly confusing, ways of reporting one Terabyte.
SI prefixs (Hard Drive) | equivalent | Binary prefixes (OS) | equivalent |
---|---|---|---|
1 TB (Terabytes) | 1 * (1000 * 1000 * 1000 * 1000) | 0.9095 TB (Terabytes) | 0.9095 * (1024 * 1024 * 1024 * 1024) |
1000 GB (Gigabytes) | 1000 * (1000 * 1000 * 1000) | 931.3 GB (Gigabytes) | 931.3 * (1024 * 1024 * 1024) |
1,000,000 MB (Megabytes) | 1,000,000 * (1000 * 1000) | 953,674.3 MB (Megabytes) | 953,674.2 * (1024 * 1024) |
1,000,000,000 KB (Kilobytes) | 1,000,000,000 * (1000) | 976,562,500 KB (Kilobytes) | 976,562,500 * (1024) |
1,000,000,000,000 B (bytes) | - | 1,000,000,000,000 B (bytes) | - |
Microsoft Windows reports disk capacity both in a decimal integer to 12 or more digits and in binary prefix units to three significant digits. The numbers will be correct, but the latter value will be mislabled with SI rather than binary prefixes (eg: shown as GB while calculated as GiB).
The capacity of an HDD can be calculated by multiplying the number of cylinders by the number of heads by the number of sectors by the number of bytes/sector (most commonly 512). Drives with the ATA interface and a capacity of eight gigabytes or more behave as if they were structured into 16383 cylinders, 16 heads, and 63 sectors, for compatibility with older operating systems. Unlike in the 1980s, the cylinder, head, sector (C/H/S) counts reported to the CPU by a modern ATA drive are no longer actual physical parameters since the reported numbers are constrained by historic operating-system interfaces and with zone bit recording the actual number of sectors varies by zone. Disks with SCSI interface address each sector with a unique integer number; the operating system remains ignorant of their head or cylinder count.
The old C/H/S scheme has been replaced by logical block addressing. In some cases, to try to "force-fit" the C/H/S scheme to large-capacity drives, the number of heads was given as 64, although no modern drive has anywhere near 32 platters.
Form factors
2½″ (8.5 mm) 6495 MB HDD,
US/UK pennies for comparison.

Before the era of PCs and small computers, hard disks were of widely varying dimensions, typically in free standing cabinets the size of washing machines (e.g. DEC RP06 Disk Drive) or designed so that dimensions enabled placement in a 19" rack (e.g. Diablo Model 31).
With increasing sales of small computers having built in floppy-disk drives (FDDs), HDDs that would fit to the FDD mountings became desirable, and this led to the evolution of the market towards drives with certain Form factors, initially derived from the sizes of 8", 5.25" and 3.5" floppy disk drives. Smaller sizes than 3.5" have emerged as popular in the marketplace and/or been decided by various industry groups.
- 8 inch: 9.5 in × 4.624 in × 14.25 in (241.3 mm × 117.5 mm × 362 mm)
In 1979, Shugart Associates' SA1000 was the first form factor compatible HDD, having the same dimensions and a compatible interface to the 8″ FDD. - 5.25 inch: 5.75 in × 1.63 in × 8 in (146.1 mm × 41.4 mm × 203 mm)
This smaller form factor, first used in an HDD by Seagate in 1980, was the same size as full height 5¼-inch diameter FDD, i.e., 3.25 inches high. This is twice as high as "half height" commonly used today; i.e., 1.63 in (41.4 mm). Most desktop models of drives for optical 120 mm disks (DVD, CD) use the half height 5¼″ dimension, but it fell out of fashion for HDDs. The Quantum Bigfoot HDD was the last to use it in the late 1990s, with “low-profile” (≈25 mm) and “ultra-low-profile” (≈20 mm) high versions. - 3.5 inch: 4 in × 1 in × 5.75 in (101.6 mm × 25.4 mm × 146 mm) = 376.77344 cm³
This smaller form factor, first used in an HDD by Rodime in 1984, was the same size as the "half height" 3½″ FDD, i.e., 1.63 inches high. Today has been largely superseded by 1-inch high “slimline” or “low-profile” versions of this form factor which is used by most desktop HDDs. - 2.5 inch: 2.75 in × 0.374–0.59 in × 3.945 in (69.85 mm × 9.5–15 mm × 100 mm) = 66.3575 cm³-104.775 cm³
This smaller form factor was introduced by PrairieTek in 1988; there is no corresponding FDD. It is widely used today for hard-disk drives in mobile devices (laptops, music players, etc.) and as of 2008 replacing 3.5 inch enterprise-class drives. It is also used in the Playstation 3 video game console. Today, the dominant height of this form factor is 9.5 mm for laptop drives, but high capacity drives used to have a height of 12.5 mm. Enterprise-class drives can have a height up to 15 mm.[31] - 1.8 inch: 54 mm × 8 mm × 71 mm = 30.672 cm³
This form factor, originally introduced by Integral Peripherals in 1993, has evolved into the ATA-7 LIF with dimensions as stated. It is increasingly used in digital audio players and subnotebooks. An original variant exists for 2–5 GB sized HDDs that fit directly into a PC card expansion slot. These became popular for their use in iPods and other HDD based MP3 players. - 1 inch: 42.8 mm × 5 mm × 36.4 mm
This form factor was introduced in 1999 as IBM's Microdrive to fit inside a CF Type II slot. Samsung calls the same form factor "1.3 inch" drive in its product literature.[32] - 0.85 inch: 24 mm × 5 mm × 32 mm
Toshiba announced this form factor in January 2004[33] for use in mobile phones and similar applications, including SD/MMC slot compatible HDDs optimized for video storage on 4G handsets. Toshiba currently sells a 4 GB (MK4001MTD) and 8 GB (MK8003MTD) version[6] and holds the Guinness World Record for the smallest hard disk drive.[34]
As of 2008, 3.5" and 2.5" hard disks dominate.
By 2009 all manufacturers had discontinued the development of new products for the 1.3-inch, 1-inch and 0.85-inch form factors due to falling prices of flash memory,[35][36]
The inch-based nickname of all these form factors usually do not indicate any actual product dimension (which are specified in millimeters for more recent form factors), but just roughly indicate a size relative to disk diameters, in the interest of historic continuity.
Other characteristics
Data transfer rate
As of 2008, a typical 7200rpm desktop hard drive has a sustained "disk-to-buffer" data transfer rate of about 70 megabytes per second.[37] This rate depends on the track location, so it will be highest for data on the outer tracks (where there are more data sectors) and lower toward the inner tracks (where there are fewer data sectors); and is generally somewhat higher for 10,000rpm drives. A current widely-used standard for the "buffer-to-computer" interface is 3.0 Gbit/s SATA, which can send about 300 megabyte/s. from the buffer to the computer, and thus is still comfortably ahead of today's disk-to-buffer transfer rates. Data transfer rate (read/write) can be measured by writing a large file to disk using special file generator tools, then reading back the file. Transfer rate can be influenced by the fragmentation of the drive and the layout of the files. For example, a single file of 10GB will be read significantly faster than 1000 files of 10MB.
Seek time
Seek time currently ranges from just under 2 ms for high-end server drives, to 15 ms for miniature drives, with the most common desktop type typically being around 9 ms.[citation needed] There has not been any significant improvement in this speed for some years. Some early PC drives used a stepper motor to move the heads, and as a result had access times as slow as 80–120 ms, but this was quickly improved by voice-coil type actuation in the late 1980s, reducing access times to around 20 ms.
Power consumption
Power consumption has become considered increasingly important, not just in mobile devices such as laptops but also in server and desktop markets. Increasing data center machine density has led to problems delivering sufficient power to devices, and getting rid of the waste heat subsequently produced, as well as environmental and electrical cost concerns (see green computing). Similar issues exist for large companies with thousands of desktop PCs. Smaller form factor drives often use less power than larger drives. One interesting development in this area is actively controlling the seek speed so that the head arrives at its destination only just in time to read the sector, rather than arriving as quickly as possible and then having to wait for the sector to come around (i.e. the rotational latency).
Audible noise
Measured in dBA, audible noise is significant for certain applications, such as PVRs, digital audio recording and quiet computers. Low noise disks typically use fluid bearings, slower rotational speeds (usually 5,400 rpm) and reduce the seek speed under load (AAM) to reduce audible clicks and crunching sounds. Drives in smaller form factors (e.g. 2.5 inch) are often quieter than larger drives.
Shock resistance
Shock resistance is especially important for mobile devices. Some laptops now include a motion sensor that parks the disk heads if the machine is dropped, hopefully before impact, to offer the greatest possible chance of survival in such an event. Maximum shock tolerance to date is 350 Gs for operating and 900 Gs for non-operating.
Access and interfaces
Hard disk drives are accessed over one of a number of bus types, including parallel ATA (P-ATA, also called IDE or EIDE), Serial ATA (SATA), SCSI, Serial Attached SCSI (SAS), and Fibre Channel. Bridge circuitry is sometimes used to connect hard disk drives to buses that they cannot communicate with natively, such as IEEE 1394, USB and SCSI.
Back in the days of the ST-506 interface, the data encoding scheme was also important. The first ST-506 disks used Modified Frequency Modulation (MFM) encoding, and transferred data at a rate of 5 megabits per second. Later on, controllers using 2,7 RLL (or just "RLL") encoding increased the transfer rate by 50%, to 7.5 megabits per second; this also increased disk capacity by fifty percent.
Many ST-506 interface disk drives were only specified by the manufacturer to run at the lower MFM data rate, while other models (usually more expensive versions of the same basic disk drive) were specified to run at the higher RLL data rate. In some cases, a disk drive had sufficient margin to allow the MFM specified model to run at the faster RLL data rate; however, this was often unreliable and was not recommended. An RLL-certified disk drive could run on a MFM controller, but with 1/3 less data capacity and speed.
Enhanced Small Disk Interface (ESDI) also supported multiple data rates (ESDI disks always used 2,7 RLL, but at 10, 15 or 20 megabits per second), but this was usually negotiated automatically by the disk drive and controller; most of the time, however, 15 or 20 megabit ESDI disk drives weren't downward compatible (i.e. a 15 or 20 megabit disk drive wouldn't run on a 10 megabit controller). ESDI disk drives typically also had jumpers to set the number of sectors per track and (in some cases) sector size.
Modern hard drives present a consistent interface to the rest of the computer, no matter what data encoding scheme is used internally. Typically a DSP in the electronics inside the hard drive takes the raw analog voltages from the read head and uses PRML and Reed–Solomon error correction[38] to decode the sector boundaries and sector data, then sends that data out the standard interface. That DSP also watches the error rate detected by error detection and correction, and performs bad sector remapping, data collection for Self-Monitoring, Analysis, and Reporting Technology, and other internal tasks.
SCSI originally had just one signaling frequency of 5 MHz for a maximum data rate of 5 megabytes/second over 8 parallel conductors, but later this was increased dramatically. The SCSI bus speed had no bearing on the disk's internal speed because of buffering between the SCSI bus and the disk drive's internal data bus; however, many early disk drives had very small buffers, and thus had to be reformatted to a different interleave (just like ST-506 disks) when used on slow computers, such as early Commodore Amiga, IBM PC compatibles and Apple Macintoshes.
ATA disks have typically had no problems with interleave or data rate, due to their controller design, but many early models were incompatible with each other and couldn't run with two devices on the same physical cable in a master/slave setup. This was mostly remedied by the mid-1990s, when ATA's specification was standardised and the details began to be cleaned up, but still causes problems occasionally (especially with CD-ROM and DVD-ROM disks, and when mixing Ultra DMA and non-UDMA devices).
Serial ATA does away with master/slave setups entirely, placing each disk on its own channel (with its own set of I/O ports) instead.
FireWire/IEEE 1394 and USB(1.0/2.0) HDDs are external units containing generally ATA or SCSI disks with ports on the back allowing very simple and effective expansion and mobility. Most FireWire/IEEE 1394 models are able to daisy-chain in order to continue adding peripherals without requiring additional ports on the computer itself. USB however, is a point to point network and doesn't allow for daisy-chaining. USB hubs are used to increase the number of available ports and are used for devices that don't require charging since the current supplied by hubs is typically lower than what's available from the built-in USB ports.
Disk interface families used in personal computers
Notable families of disk interfaces include:
- Historical bit serial interfaces — connect a hard disk drive (HDD) to a hard disk controller (HDC) with two cables, one for control and one for data. (Each drive also has an additional cable for power, usually connecting it directly to the power supply unit). The HDC provided significant functions such as serial/parallel conversion, data separation, and track formatting, and required matching to the drive (after formatting) in order to assure reliability. Each control cable could serve two or more drives, while a dedicated (and smaller) data cable served each drive.
- ST506 used MFM (Modified Frequency Modulation) for the data encoding method.
- ST412 was available in either MFM or RLL (Run Length Limited) encoding variants.
- Enhanced Small Disk Interface (ESDI) was an interface developed by Maxtor to allow faster communication between the processor and the disk than MFM or RLL.
- Modern bit serial interfaces — connect a hard disk drive to a host bus interface adapter (today typically integrated into the "south bridge") with one data/control cable. (As for historical bit serial interfaces above, each drive also has an additional power cable, usually direct to the power supply unit.)
- Fibre Channel (FC), is a successor to parallel SCSI interface on enterprise market. It is a serial protocol. In disk drives usually the Fibre Channel Arbitrated Loop (FC-AL) connection topology is used. FC has much broader usage than mere disk interfaces, it is the cornerstone of storage area networks (SANs). Recently other protocols for this field, like iSCSI and ATA over Ethernet have been developed as well. Confusingly, drives usually use copper twisted-pair cables for Fibre Channel, not fibre optics. The latter are traditionally reserved for larger devices, such as servers or disk array controllers.
- Serial ATA (SATA). The SATA data cable has one data pair for differential transmission of data to the device, and one pair for differential receiving from the device, just like EIA-422. That requires that data be transmitted serially. Similar differential signaling system is used in RS485, LocalTalk, USB, Firewire, and differential SCSI.
- Serial Attached SCSI (SAS). The SAS is a new generation serial communication protocol for devices designed to allow for much higher speed data transfers and is compatible with SATA. SAS uses a mechanically identical data and power connector to standard 3.5" SATA1/SATA2 HDDs, and many server-oriented SAS RAID controllers are also capable of addressing SATA hard drives. SAS uses serial communication instead of the parallel method found in traditional SCSI devices but still uses SCSI commands.
- Word serial interfaces — connect a hard disk drive to a host bus adapter (today typically integrated into the "south bridge") with one cable for combined data/control. (As for all bit serial interfaces above, each drive also has an additional power cable, usually direct to the power supply unit.) The earliest versions of these interfaces typically had a 8 bit parallel data transfer to/from the drive, but 16 bit versions became much more common, and there are 32 bit versions. Modern variants have serial data transfer. The word nature of data transfer makes the design of a host bus adapter significantly simpler than that of the precursor HDD controller.
- Integrated Drive Electronics (IDE), later renamed to ATA, with the alias P-ATA ("parallel ATA") retroactively added upon introduction of the new variant Serial ATA. The original name reflected the innovative integration of HDD controller with HDD itself, which was not found in earlier disks. Moving the HDD controller from the interface card to the disk drive helped to standardize interfaces, and to reduce the cost and complexity. The 40 pin IDE/ATA connection transfers 16 bits of data at a time on the data cable. The data cable was originally 40 conductor, but later higher speed requirements for data transfer to and from the hard drive led to an "ultra DMA" mode, known as UDMA. Progressively faster versions of this standard ultimately added the requirement for an 80 conductor variant of the same cable; where half of the conductors provides grounding necessary for enhanced high-speed signal quality by reducing cross talk. The interface for 80 conductor only has 39 pins, the missing pin acting as a key to prevent incorrect insertion of the connector to an incompatible socket, a common cause of disk and controller damage.
- EIDE was an unofficial update (by Western Digital) to the original IDE standard, with the key improvement being the use of direct memory access (DMA) to transfer data between the disk and the computer without the involvement of the CPU, an improvement later adopted by the official ATA standards. By directly transferring data between memory and disk, DMA eliminates the need for the CPU to copy byte per byte, therefore allowing it to process other tasks while the data transfer occurs.
- Small Computer System Interface (SCSI), originally named SASI for Shugart Associates System Interface, was an early competitor of ESDI. SCSI disks were standard on servers, workstations, Commodore Amiga and Apple Macintosh computers through the mid-90s, by which time most models had been transitioned to IDE (and later, SATA) family disks. Only in 2005 did the capacity of SCSI disks fall behind IDE disk technology, though the highest-performance disks are still available in SCSI and Fibre Channel only. The length limitations of the data cable allows for external SCSI devices. Originally SCSI data cables used single ended (common mode) data transmission, but server class SCSI could use differential transmission, either low voltage differential (LVD) or high voltage differential (HVD). ("Low" and "High" voltages for differential SCSI are relative to SCSI standards and do not meet the meaning of low voltage and high voltage as used in general electrical engineering contexts, as apply e.g. to statutory electrical codes; both LVD and HVD use low voltage signals (3.3 V and 5 V respectively) in general terminology.)
Acronym or abbreviation | Meaning | Description |
---|---|---|
SASI | Shugart Associates System Interface | Historical predecessor to SCSI. |
SCSI | Small Computer System Interface | Bus oriented that handles concurrent operations. |
SAS | Serial Attached SCSI | Improvement of SCSI, uses serial communication instead of parallel. |
ST-506 | Seagate Technology | Historical Seagate interface. |
ST-412 | Seagate Technology | Historical Seagate interface (minor improvement over ST-506). |
ESDI | Enhanced Small Disk Interface | Historical; backwards compatible with ST-412/506, but faster and more integrated. |
ATA | Advanced Technology Attachment | Successor to ST-412/506/ESDI by integrating the disk controller completely onto the device. Incapable of concurrent operations. |
SATA | Serial ATA | Modification of ATA, uses serial communication instead of parallel. |
Integrity


Due to the extremely close spacing between the heads and the disk surface, any contamination of the read-write heads or platters can lead to a head crash — a failure of the disk in which the head scrapes across the platter surface, often grinding away the thin magnetic film and causing data loss. Head crashes can be caused by electronic failure, a sudden power failure, physical shock, wear and tear, corrosion, or poorly manufactured platters and heads.
The HDD's spindle system relies on air pressure inside the enclosure to support the heads at their proper flying height while the disk rotates. Hard disk drives require a certain range of air pressures in order to operate properly. The connection to the external environment and pressure occurs through a small hole in the enclosure (about 0.5 mm in diameter), usually with a carbon filter on the inside (the breather filter).[citation needed] If the air pressure is too low, then there is not enough lift for the flying head, so the head gets too close to the disk, and there is a risk of head crashes and data loss. Specially manufactured sealed and pressurized disks are needed for reliable high-altitude operation, above about 3,000 m (10,000 feet). Note that modern commercial aircraft have a pressurized cabin, whose pressure altitude does not normally exceed 2,600 m(8,500 feet) - thus, ordinary hard drives can safely be used in flight.[citation needed] Modern disks include temperature sensors and adjust their operation to the operating environment. Breather holes can be seen on all disk drives — they usually have a sticker next to them, warning the user not to cover the holes. The air inside the operating drive is constantly moving too, being swept in motion by friction with the spinning platters. This air passes through an internal recirculation (or "recirc") filter to remove any leftover contaminants from manufacture, any particles or chemicals that may have somehow entered the enclosure, and any particles or outgassing generated internally in normal operation. Very high humidity for extended periods can corrode the heads and platters.
For giant magnetoresistive (GMR) heads in particular, a minor head crash from contamination (that does not remove the magnetic surface of the disk) still results in the head temporarily overheating, due to friction with the disk surface, and can render the data unreadable for a short period until the head temperature stabilizes (so called "thermal asperity", a problem which can partially be dealt with by proper electronic filtering of the read signal).
Actuation of moving arm
The hard drive's electronics control the movement of the actuator and the rotation of the disk, and perform reads and writes on demand from the disk controller. Feedback of the drive electronics is accomplished by means of special segments of the disk dedicated to servo feedback. These are either complete concentric circles (in the case of dedicated servo technology), or segments interspersed with real data (in the case of embedded servo technology). The servo feedback optimizes the signal to noise ratio of the GMR sensors by adjusting the voice-coil of the actuated arm. The spinning of the disk also uses a servo motor. Modern disk firmware is capable of scheduling reads and writes efficiently on the platter surfaces and remapping sectors of the media which have failed.
Landing zones and load/unload technology
Modern HDDs prevent power interruptions or other malfunctions from landing its heads in the data zone by parking the heads either in a landing zone or by unloading (i.e., load/unload) the heads. Some early PC HDDs did not park the heads and they could land on data.
A landing zone is an area of the platter usually near its inner diameter (ID), where no data is stored. This area is called the Contact Start/Stop (CSS) zone. Disks are designed such that either a spring or, more recently, rotational inertia in the platters is used to park the heads in the case of unexpected power loss. In this case, the spindle motor temporarily acts as a generator, providing power to the actuator.
Spring tension from the head mounting constantly pushes the heads towards the platter. While the disk is spinning, the heads are supported by an air bearing and experience no physical contact or wear. In CSS drives the sliders carrying the head sensors (often also just called heads) are designed to survive a number of landings and takeoffs from the media surface, though wear and tear on these microscopic components eventually takes its toll. Most manufacturers design the sliders to survive 50,000 contact cycles before the chance of damage on startup rises above 50%. However, the decay rate is not linear: when a disk is younger and has had fewer start-stop cycles, it has a better chance of surviving the next startup than an older, higher-mileage disk (as the head literally drags along the disk's surface until the air bearing is established). For example, the Seagate Barracuda 7200.10 series of desktop hard disks are rated to 50,000 start-stop cycles, in other words no failures attributed to the head-platter interface were seen before at least 50,000 start-stop cycles during testing.[39]
Around 1995 IBM pioneered a technology where a landing zone on the disk is made by a precision laser process (Laser Zone Texture = LZT) producing an array of smooth nanometer-scale "bumps" in a landing zone,[40] thus vastly improving stiction and wear performance. This technology is still largely in use today (2008), predominantly in desktop and enterprise (3.5 inch) drives. In general, CSS technology can be prone to increased stiction (the tendency for the heads to stick to the platter surface), e.g. as a consequence of increased humidity. Excessive stiction can cause physical damage to the platter and slider or spindle motor.
Load/Unload technology relies on the heads being lifted off the platters into a safe location, thus eliminating the risks of wear and stiction altogether. The first HDD RAMAC and most early disk drives used complex mechanisms to load and unload the heads. Modern HDDs use ramp loading, first introduced by Memorex in 1967,[41] to load/unload onto plastic "ramps" near the outer disk edge.
All HDDs today still use one of these two technologies listed above. Each has a list of advantages and drawbacks in terms of loss of storage area on the disk, relative difficulty of mechanical tolerance control, non-operating shock robustness, cost of implementation, etc.
Addressing shock robustness, IBM also created a technology for their ThinkPad line of laptop computers called the Active Protection System. When a sudden, sharp movement is detected by the built-in accelerometer in the Thinkpad, internal hard disk heads automatically unload themselves to reduce the risk of any potential data loss or scratch defects. Apple later also utilized this technology in their PowerBook, iBook, MacBook Pro, and MacBook line, known as the Sudden Motion Sensor. Sony,[42] HP with their HP 3D DriveGuard[43] and Toshiba[44] have released similar technology in their notebook computers.
This accelerometer based shock sensor has also been used for building cheap earthquake sensor networks.[45]
Disk failures and their metrics

Most major hard disk and motherboard vendors now support S.M.A.R.T. (Self-Monitoring, Analysis, and Reporting Technology), which measures drive characteristics such as temperature, spin-up time, data error rates, etc. Certain trends and sudden changes in these parameters are thought to be associated with increased likelihood of drive failure and data loss.
However, not all failures are predictable. Normal use eventually can lead to a breakdown in the inherently fragile device, which makes it essential for the user to periodically back up the data onto a separate storage device. Failure to do so will lead to the loss of data. While it may sometimes be possible to recover lost information, it is normally an extremely costly procedure, and it is not possible to guarantee success. A 2007 study published by Google suggested very little correlation between failure rates and either high temperature or activity level; however, the correlation between manufacturer/model and failure rate was relatively strong. Statistics in this matter is kept highly secret by most entities. Google did not publish the manufacturer's names along with their respective failure rates,[46] though they have since revealed that they use Hitachi Deskstar drives in some of their servers.[47] While several S.M.A.R.T. parameters have an impact on failure probability, a large fraction of failed drives do not produce predictive S.M.A.R.T. parameters.[46] S.M.A.R.T. parameters alone may not be useful for predicting individual drive failures.[46]
A common misconception is that a colder hard drive will last longer than a hotter hard drive. The Google study seems to imply the reverse -- "lower temperatures are associated with higher failure rates". Hard drives with S.M.A.R.T.-reported average temperatures below 27 °C had failure rates worse than hard drives with the highest reported average temperature of 50 °C, failure rates at least twice as high as the optimum S.M.A.R.T.-reported temperature range of 36 °C to 47 °C.[46]
SCSI, SAS and FC drives are typically more expensive and are traditionally used in servers and disk arrays, whereas inexpensive ATA and SATA drives evolved in the home computer market and were perceived to be less reliable. This distinction is now becoming blurred.
The mean time between failures (MTBF) of SATA drives is usually about 600,000 hours (some drives such as Western Digital Raptor have rated 1.2 million hours MTBF), while SCSI drives are rated for upwards of 1.5 million hours.[citation needed] However, independent research indicates that MTBF is not a reliable estimate of a drive's longevity.[48] MTBF is conducted in laboratory environments in test chambers and is an important metric to determine the quality of a disk drive before it enters high volume production. Once the drive product is in production, the more valid metric is annualized failure rate (AFR).[citation needed] AFR is the percentage of real-world drive failures after shipping.
SAS drives are comparable to SCSI drives, with high MTBF and high reliability.[citation needed]
Enterprise S-ATA drives designed and produced for enterprise markets, unlike standard S-ATA drives, have reliability comparable to other enterprise class drives.[49][50]
Typically enterprise drives (all enterprise drives, including SCSI, SAS, enterprise SATA and FC) experience between 0.70%-0.78% annual failure rates from the total installed drives. [citation needed]
Eventually all mechanical hard disk drives fail. And thus the strategy to mitigate loss of data is to have redundancy in some form, like RAID and backup. RAID should never be relied on as backup, as RAID controllers also break down, making the disks inaccessible. Following a backup strategy, for example - daily differential and weekly full backups, is the only sure way to prevent data loss.
Manufacturers


The technological resources and know-how required for modern drive development and production mean that as of 2009, virtually all of the world's HDDs are manufactured by just five large companies: Seagate, Western Digital, Hitachi (which owns the former disk manufacturing division of IBM), Samsung, and Toshiba.
Dozens of former HDD manufacturers have gone out of business, merged, or closed their HDD divisions; as capacities and demand for products increased, profits became hard to find, and the market underwent significant consolidation in the late 1980s and late 1990s. The first notable casualty of the business in the PC era was Computer Memories Inc. or CMI; after an incident with faulty 20 MB AT disks in 1985,[51] CMI's reputation never recovered, and they exited the HDD business in 1987. Another notable failure was MiniScribe, who went bankrupt in 1990 after it was found that they had engaged in accounting fraud and inflated sales numbers for several years. Many other smaller companies (like Kalok, Microscience, LaPine, Areal, Priam and PrairieTek) also did not survive the shakeout, and had disappeared by 1993; Micropolis was able to hold on until 1997, and JTS, a relative latecomer to the scene, lasted only a few years and was gone by 1999, after attempting to manufacture HDDs in India. Their claim to fame was creating a new 3″ form factor drive for use in laptops. Quantum and Integral also invested in the 3″ form factor; but eventually ceased support as this form factor failed to catch on. Rodime was also an important manufacturer during the 1980s, but stopped making disks in the early 1990s amid the shakeout and now concentrates on technology licensing; they hold a number of patents related to 3.5-inch form factor HDDs.
![]() |
- 1988: Tandon Corporation sold its disk manufacturing division to Western Digital (WDC), which was then a well-known controller designer.[52]
- 1989: Seagate Technology bought Control Data's high-end disk business, as part of CDC's exit from hardware manufacturing.
- 1990: Maxtor buys MiniScribe out of bankruptcy, making it the core of its low-end disk division.
- 1994: Quantum bought DEC's storage division, giving it a high-end disk range to go with its more consumer-oriented ProDrive range, as well as the DLT tape drive range.
- 1995: Conner Peripherals, which was founded by one of Seagate Technology's co-founders along with personnel from MiniScribe, announces a merger with Seagate, which was completed in early 1996.
- 1996: JTS merges with Atari, allowing JTS to bring its disk range into production. Atari was sold to Hasbro in 1998, while JTS itself went bankrupt in 1999.
- 2000: Quantum sells its disk division to Maxtor to concentrate on tape drives and backup equipment.
- 2003: Following the controversy over mass failures of its Deskstar 75GXP range, HDD pioneer IBM sold the majority of its disk division to Hitachi, who renamed it Hitachi Global Storage Technologies (HGST).
- 2003: Western Digital purchased Read-Rite Corp, which makes recording heads used on disk drive platters, for $95.4 million in cash.
- December 21, 2005: Seagate and Maxtor announced an agreement under which Seagate would acquire Maxtor in an all stock transaction valued at $1.9 billion. The acquisition was approved by the appropriate regulatory bodies, and closed on May 19, 2006.
- 2007
- July: Western Digital (WDC) acquires Komag U.S.A, a thin-film media manufacturer, for USD 1 Billion.[53]
- 2009: Excelstor no longer offering HDDs
- 2009: Toshiba acquires Fujitsu disk division[54]
Sales
In the year 2007 516.2 million hard disks were sold .[55]
See also
- Automatic Acoustic Management
- Click of death
- Computer History Museum's HDD Working Group Website
- Data erasure
- Disk formatting
- du (Unix disk usage program)
- Drive mapping
- External hard disk drive
- History of hard disk drives
- Hybrid drive
- IBM 305 RAMAC
- Solid-state drive
- Spintronics
References
- ^ Other terms use to describe hard disk drives include disk drive, disk file, DASD (Direct Access Storage Device), fixed disk, CKD disk and Winchester Disk Drive (after the IBM 3340).
- ^ http://www.webopedia.com/TERM/h/hard_disk.html
- ^ http://searchstorage.techtarget.com/sDefinition/0,,sid5_gci213993,00.html
- ^ How Hard Disks Work, howstuffworks.com
- ^ In the 1990s there was a partial return to the use of removable hard disks, such as the Iomega Jaz and Rev drives and disks and the SyQuest SyJet and Sparq drives and disks, and the Castlewood Orb drive and disk, among other models, but as of 2009 these are mostly defunct.
- ^ [1] IBM 350 disk storage unit
- ^ "IBM OEM MR Head | Technology | The era of giant magnetoresistive heads". Hitachigst.com. 2001-08-27. Retrieved 2009-03-13.
- ^ Brian Hayes, Terabyte Territory, American Scientist, Vol 90 No 3 (May-June 2002) p. 212
- ^ "Press Releases December 14, 2004". Toshiba. Retrieved 2009-03-13.
- ^ "Seagate Momentus 2½" HDDs per webpage Jan 2008". Seagate.com. 2008-10-24. Retrieved 2009-03-13.
- ^ "Seagate Baracuda 3½" HDDs per webpage Jan 2008". Seagate.com. Retrieved 2009-03-13.
- ^ "Western Digital Scorpio 2½" and Greenpower 3½" HDDs per quarterly conference, July 2007". Wdc.com. Retrieved 2009-03-13.
- ^ Storage Review - Error Correcting Code
- ^ Murph, Darren (2009-01-26). "Western Digital's 2TB Caviar Green HDD on sale in Australia". Engadget.com. Retrieved 2009-03-13.
- ^ PC Magazine comparison of 136 desktops shows 60 in this HDD capacity range with 50 larger and 26 smaller capacities [2]
- ^ a b Seagate Cheetah 15K.5[3]
- ^ Walter, Chip (July 25, 2005). "Kryder's Law". Scientific American. Verlagsgruppe Georg von Holtzbrinck GmbH. Retrieved 2006-10-29.
- ^ "Seagate Outlines the Future of Storage :: Articles :: www.hardwarezone.com". www.hardwarezone.com<!. 2006-01-27. Retrieved 2009-03-13.
- ^ "Hitachi's 7K1000 Terabyte Hard Drive". Tomshardware.com. Retrieved 2009-03-13.
- ^ "Seagate, Samsung Begin to Ship 1 TB Desktop Hard Drives". Dailytech.com. Retrieved 2009-03-13.
- ^ "WD Caviar GP: The "Green" 1 TB Drive". Tomshardware.com. Retrieved 2009-03-13.
- ^ "Translation result for http://www.watch.impress.co.jp/akiba/hotline/20081227/etc_seagate.html". Babelfish.yahoo.com. Retrieved 2009-03-13.
{{cite web}}
: External link in
(help)|title=
- ^ Seagate Elite 47, shipped 12/97 per 1998 Disk/Trend Report - Rigid Disk Drives
- ^ Quantum Bigfoot TS, shipped 10/98 per 1999 Disk/Trend Report - Rigid Disk Drives
- ^ The Quantum Bigfoot TS used a maximum of 3 platters, other earlier and lower capacity product used up to 4 platters in a 5.25″ HH form factor, e.g. Microscience HH1090 circa 1989.
- ^ Murphy, David. "Western Digital Launches World-First 2TB Hard Drive". PC World. Retrieved 2009-01-27.
- ^ "Hitachi announces 500GB laptop drive". 080103 http://www.macworld.com
- ^ "Toshiba Storage Solutions - 1.8-Inch SATA - 250GB HDDs".
- ^ "SDK Starts Shipments of 1.3-Inch PMR-Technology-Based HD Media". Sdk.co.jp. 2008-01-10. Retrieved 2009-03-13.
- ^ "Toshiba's World Smallest Hard Disk Drive". Toshibastorage.com. Retrieved 2009-03-13.
- ^ "One Drive, Multiple Applications - Tom's Hardware : WD's New Raptor Drive Is a Bird of Prey!". Tomshardware.com. 2008-04-21. Retrieved 2009-03-13.
- ^ 1.3″ HDD Product Specification, Samsung, 2008
- ^ Toshiba's 0.85-inch HDD is set to bring multi-gigabyte capacities to small, powerful digital products, Toshiba press release, January 8, 2004
- ^ Toshiba enters Guinness World Records Book with the world's smallest hard disk drive, Toshiba press release, March 16, 2004
- ^ Flash price fall shakes HDD market, EETimes Asia, August 1, 2007.
- ^ In 2008 Samsung introduced the 1.3-inch SpinPoint A1 HDD but by March 2009 the family was listed as End Of Life Products and new new 1.3-inch models were not available in this size.
- ^ http://wdc.com/en/library/sata/2879-701277.pdf
- ^ "Reed Solomon Codes - Introduction"
- ^ Barracuda 7200.10 Serial ATA Product Manual
- ^ [4] IEEE Trans. Magn.
- ^ Pugh et al.; "IBM's 360 and Early 370 Systems"; MIT Press, 1991, pp.270
- ^ "Sony | For Business | VAIO SMB". B2b.sony.com. Retrieved 2009-03-13.
- ^ [5]
- ^ Toshiba HDD Protection measures.
- ^ "Quake-Catcher Network". 090128 qcn.stanford.edu
- ^ a b c d
Eduardo Pinheiro, Wolf-Dietrich Weber and Luiz André Barroso (2007). "Failure Trends in a Large Disk Drive Population". USENIX Conference on File and Storage Technologies. 5th USENIX Conference on File and Storage Technologies (FAST 2007). Retrieved 2008-09-15.
{{cite conference}}
: External link in
(help); Unknown parameter|conferenceurl=
|booktitle=
ignored (|book-title=
suggested) (help); Unknown parameter|conferenceurl=
ignored (|conference-url=
suggested) (help); Unknown parameter|month=
ignored (help) - ^ http://news.cnet.com/8301-1001_3-10209580-92.html
- ^ "Everything You Know About Disks Is Wrong". StorageMojo. February 20, 2007. Retrieved 2007-08-29.
- ^ "Differences between an Enterprise-Class HDD and a Desktop-Class HDD". Synology.com. 2008-09-04. Retrieved 2009-03-13.
- ^ Intel Whitepaper on Enterprise-class versus Desktop-class Hard Drives
- ^ Apparently the CMI disks suffered from a higher soft error rate than IBM's other suppliers (Seagate and MiniScribe) but the bugs in Microsoft's DOS Operating system may have turned these recoverable errors into hard failures. At some point, possibly MS-DOS 3.0, soft errors were reported as disk hard errors and a subsequent Microsoft patch turned soft errors into corrupted memory with unpredictable results ("crashes"). MS-DOS 3.3 apparently resolved this series of problems but by that time it was too late for CMI. See also, "IBM and CMI in Joint Effort to Rehab AT Hard-Disk Rejects", PC Week, v.2 n.11, p.1, March 19, 1985
- ^
"COMPANY NEWS; Tandon Sells Disk Drive Unit". The New York Times. 1988-03-09. Retrieved 2008-02-22.
{{cite web}}
: Cite has empty unknown parameter:|coauthors=
(help) - ^ "Western Digital buys Komag for $1 Billion". Retrieved 2007-08-18.
- ^ "Fujitsu to Split Off HDD Business in Reorganization" (PDF). Retrieved 2009-06-02.
- ^ Hard drive industry shrugs off economic concerns, posts double digit growth rates, accessed at 11 january 2009
External links
