Light: Difference between revisions
mNo edit summary |
|||
Line 23: | Line 23: | ||
Although some people speak of the "velocity of light", the word ''[[velocity]]'' should be reserved for [[vector (spatial)|vector]] quantities, that is, those with both [[magnitude]] and [[direction]]. The speed of light is a [[scalar]] quantity, having only magnitude and no direction, and therefore ''speed'' is the correct term. |
Although some people speak of the "velocity of light", the word ''[[velocity]]'' should be reserved for [[vector (spatial)|vector]] quantities, that is, those with both [[magnitude]] and [[direction]]. The speed of light is a [[scalar]] quantity, having only magnitude and no direction, and therefore ''speed'' is the correct term. |
||
Some scholars have conjectured that the speed of light was first known in [[India]], based on a statement by [[Sayana]] (c. [[1315]]-[[1387]]). [[Max Muller]]'s translation of Sayana's commentary on the [[Rig Veda]] states: "Thus it is remembered: [O Sun] you who traverse 2202 yojanas in half a nimesa." This corresponds to a speed of about 302,073 km/sec, which is close to the speed of light (299,792 km/sec). This statement also occurs in [[Bhatta Bhaskara]]'s earlier commentary on the ''[[Taittiriya Brahmana]]''. There is still much debate on whether Sayana was estimating the speed of light or overestimating the speed of the [[Sun]], since he didn't make it clear which he was referring to, nor did he give any methods for his estimate. |
Some scholars have conjectured that the speed of light was first known in [[India]], based on a statement by [[Sayana]] (c. [[1315]]-[[1387]]). [[Max Muller]]'s translation of Sayana's commentary on the [[Rig Veda]] states: "Thus it is remembered: [O Sun] you who traverse 2202 yojanas in half a nimesa." This corresponds to a speed of about 302,073 km/sec, which is close to the speed of light (299,792 km/sec). This statement also occurs in [[Bhatta Bhaskara]]'s earlier commentary on the ''[[Taittiriya Brahmana]]''. There is still much debate on whether Sayana was estimating the speed of light or overestimating the speed of the [[Sun]], since he didn't make it clear which he was referring to, nor did he give any methods for his estimate. {{inote|S. Kak, 1998}} |
||
The speed of light has been measured many times, by many physicists. The best early measurement in Europe is by [[Ole Rømer]], a Danish physicist, in [[1676]]. By observing the motions of [[Jupiter (planet)|Jupiter]] and one of its [[natural satellite|moon]]s, [[Io (moon)|Io]], with a [[telescope]], and noting discrepancies in the apparent period of Io's orbit, Rømer calculated that |
The speed of light has been measured many times, by many physicists. The best early measurement in Europe is by [[Ole Rømer]], a Danish physicist, in [[1676]]. By observing the motions of [[Jupiter (planet)|Jupiter]] and one of its [[natural satellite|moon]]s, [[Io (moon)|Io]], with a [[telescope]], and noting discrepancies in the apparent period of Io's orbit, Rømer calculated that |
Revision as of 22:00, 22 March 2006
Light is electromagnetic radiation with a wavelength that is visible to the eye (visible light) or, in a technical or scientific context, electromagnetic radiation of any wavelength. The three basic dimensions of light (i.e., all electromagnetic radiation) are:
- Intensity (or brilliance or amplitude), which is related to the human perception of brightness of the light,
- Frequency (or wavelength), perceived by humans as the color of the light, and
- Polarization (or angle of vibration), which is not perceptible by humans under ordinary circumstances.
Due to wave-particle duality, light simultaneously exhibits properties of both waves and particles. The precise nature of light is one of the key questions of modern physics.
Visible electromagnetic radiation
Visible light is the portion of the electromagnetic spectrum between the frequencies of 380 THz (3.8×1014 hertz) and 750 THz (7.5×1014 hertz). The speed (), frequency ( or ), and wavelength () of a wave obey the relation:
Because the speed of light in a vacuum is fixed, visible light can also be characterised by its wavelength of between 400 nanometres (abbreviated 'nm') and 800 nm (in a vacuum).
Light entering the eye is absorbed by light-sensitive pigments within the rod cells and cone cells in the retina, triggering a cascade of events that creates electrical nerve impulses that travel through the optic nerve to the brain, producing vision.
Speed of light
Although some people speak of the "velocity of light", the word velocity should be reserved for vector quantities, that is, those with both magnitude and direction. The speed of light is a scalar quantity, having only magnitude and no direction, and therefore speed is the correct term.
Some scholars have conjectured that the speed of light was first known in India, based on a statement by Sayana (c. 1315-1387). Max Muller's translation of Sayana's commentary on the Rig Veda states: "Thus it is remembered: [O Sun] you who traverse 2202 yojanas in half a nimesa." This corresponds to a speed of about 302,073 km/sec, which is close to the speed of light (299,792 km/sec). This statement also occurs in Bhatta Bhaskara's earlier commentary on the Taittiriya Brahmana. There is still much debate on whether Sayana was estimating the speed of light or overestimating the speed of the Sun, since he didn't make it clear which he was referring to, nor did he give any methods for his estimate. Template:Inote
The speed of light has been measured many times, by many physicists. The best early measurement in Europe is by Ole Rømer, a Danish physicist, in 1676. By observing the motions of Jupiter and one of its moons, Io, with a telescope, and noting discrepancies in the apparent period of Io's orbit, Rømer calculated that light takes about 18 minutes to traverse the diameter of Earth's orbit. Had he known the diameter of the orbit in kilometers (which he didn't) he would have deduced a speed of 227,000 kilometres per second (approximately 141,050 miles per second).
The first successful measurement of the speed of light in Europe using an earthbound apparatus was carried out by Hippolyte Fizeau in 1849. Fizeau directed a beam of light at a mirror several thousand metres away, and placed a rotating cog wheel in the path of the beam from the source to the mirror and back again. At a certain rate of rotation, the beam could pass through one gap in the wheel on the way out and the next gap on the way back. Knowing the distance to the mirror, the number of teeth on the wheel, and the rate of rotation, Fizeau measured the speed of light as 313,000 kilometres per second.
Léon Foucault used rotating mirrors to obtain a value of 298,000 km/s (about 185,000 miles/s) in 1862. Albert A. Michelson conducted experiments on the speed of light from 1877 until his death in 1931. He refined Foucault's results in 1926 using improved rotating mirrors to measure the time it took light to make a round trip from Mt. Wilson to Mt. San Antonio in California. The precise measurements yielded a speed of 186,285 mile/s (299,796 km/s [1,079,265,600 km/h]). In daily use, the figures are rounded off to 300,000 km/s and 186,000 miles/s.
Refraction
All light propagates at a finite speed. Even moving observers always measure the same value of c, the speed of light in vacuum, as c = 299,792,458 metres per second (186,282.397 miles per second). When light passes through a transparent substance, such as air, water or glass, its speed is reduced, and it undergoes refraction. The reduction of the speed of light in a denser material can be indicated by the refractive index, n, which is defined as:
Thus, n = 1 in a vacuum and n > 1 in matter.
When a beam of light enters a medium from vacuum or another medium, it keeps the same frequency and changes its wavelength. If the incident beam is not orthogonal to the edge between the media, the direction of the beam will change. Refraction of light by lenses is used to focus light in magnifying glasses, spectacles and contact lenses, microscopes and refracting telescopes.
Optics
The study of light and the interaction of light and matter is termed optics. The observation and study of optical phenomena such as rainbows offers many clues as to the nature of light as well as much enjoyment.
Color and wavelengths
The different wavelengths are detected by the human eye and then interpreted by the brain as colors, ranging from red at the longest wavelengths of about 700 nm. (lowest frequencies) to violet at the shortest wavelengths of about 400 nm. (highest frequencies). The intervening frequencies are seen as orange, yellow, green, cyan, blue, and, conventionally, indigo.

The wavelengths of the electromagnetic spectrum immediately outside the range that the human eye is able to perceive are called ultraviolet (UV) at the short wavelength (high frequency) end and infrared (IR) at the long wavelength (low frequency) end. Some animals, such as bees, can see UV radiation while others, such as pit viper snakes, can see infrared light.
UV radiation is not normally directly perceived by humans except in a very delayed fashion, as overexposure of the skin to UV light can cause sunburn, or skin cancer, and underexposure can cause vitamin D deficiency. However, because UV is a higher frequency radiation than visible light, it very easily can cause materials to fluoresce visible light.
Cameras that can detect IR and convert it to light are called, depending on their application, night-vision cameras or infrared cameras. These are different from image intensifier cameras, which only amplify available visible light.
When intense radiation (of any frequency) is absorbed in the skin, it causes heating which can be felt. Since hot objects are strong sources of infrared radiation, IR radiation is commonly associated with this sensation. Any intense radiation that can be absorbed in the skin will have the same effect, however.
Measurement of light
The following quantities and units are used to measure the quantity or "brightness" of light.
Quantity | Unit | Dimension [nb 1] |
Notes | ||
---|---|---|---|---|---|
Name | Symbol[nb 2] | Name | Symbol | ||
Luminous energy | Qv[nb 3] | lumen second | lm⋅s | T⋅J | The lumen second is sometimes called the talbot. |
Luminous flux, luminous power | Φv[nb 3] | lumen (= candela steradian) | lm (= cd⋅sr) | J | Luminous energy per unit time |
Luminous intensity | Iv | candela (= lumen per steradian) | cd (= lm/sr) | J | Luminous flux per unit solid angle |
Luminance | Lv | candela per square metre | cd/m2 (= lm/(sr⋅m2)) | L−2⋅J | Luminous flux per unit solid angle per unit projected source area. The candela per square metre is sometimes called the nit. |
Illuminance | Ev | lux (= lumen per square metre) | lx (= lm/m2) | L−2⋅J | Luminous flux incident on a surface |
Luminous exitance, luminous emittance | Mv | lumen per square metre | lm/m2 | L−2⋅J | Luminous flux emitted from a surface |
Luminous exposure | Hv | lux second | lx⋅s | L−2⋅T⋅J | Time-integrated illuminance |
Luminous energy density | ωv | lumen second per cubic metre | lm⋅s/m3 | L−3⋅T⋅J | |
Luminous efficacy (of radiation) | K | lumen per watt | lm/W | M−1⋅L−2⋅T3⋅J | Ratio of luminous flux to radiant flux |
Luminous efficacy (of a source) | η[nb 3] | lumen per watt | lm/W | M−1⋅L−2⋅T3⋅J | Ratio of luminous flux to power consumption |
Luminous efficiency, luminous coefficient | V | 1 | Luminous efficacy normalized by the maximum possible efficacy | ||
See also: |
- ^ The symbols in this column denote dimensions; "L", "T" and "J" are for length, time and luminous intensity respectively, not the symbols for the units litre, tesla and joule.
- ^ Standards organizations recommend that photometric quantities be denoted with a subscript "v" (for "visual") to avoid confusion with radiometric or photon quantities. For example: USA Standard Letter Symbols for Illuminating Engineering USAS Z7.1-1967, Y10.18-1967
- ^ a b c Alternative symbols sometimes seen: W for luminous energy, P or F for luminous flux, and ρ for luminous efficacy of a source.
Quantity | Unit | Dimension | Notes | ||
---|---|---|---|---|---|
Name | Symbol[nb 1] | Name | Symbol | ||
Radiant energy | Qe[nb 2] | joule | J | M⋅L2⋅T−2 | Energy of electromagnetic radiation. |
Radiant energy density | we | joule per cubic metre | J/m3 | M⋅L−1⋅T−2 | Radiant energy per unit volume. |
Radiant flux | Φe[nb 2] | watt | W = J/s | M⋅L2⋅T−3 | Radiant energy emitted, reflected, transmitted or received, per unit time. This is sometimes also called "radiant power", and called luminosity in Astronomy. |
Spectral flux | Φe,ν[nb 3] | watt per hertz | W/Hz | M⋅L2⋅T −2 | Radiant flux per unit frequency or wavelength. The latter is commonly measured in W⋅nm−1. |
Φe,λ[nb 4] | watt per metre | W/m | M⋅L⋅T−3 | ||
Radiant intensity | Ie,Ω[nb 5] | watt per steradian | W/sr | M⋅L2⋅T−3 | Radiant flux emitted, reflected, transmitted or received, per unit solid angle. This is a directional quantity. |
Spectral intensity | Ie,Ω,ν[nb 3] | watt per steradian per hertz | W⋅sr−1⋅Hz−1 | M⋅L2⋅T−2 | Radiant intensity per unit frequency or wavelength. The latter is commonly measured in W⋅sr−1⋅nm−1. This is a directional quantity. |
Ie,Ω,λ[nb 4] | watt per steradian per metre | W⋅sr−1⋅m−1 | M⋅L⋅T−3 | ||
Radiance | Le,Ω[nb 5] | watt per steradian per square metre | W⋅sr−1⋅m−2 | M⋅T−3 | Radiant flux emitted, reflected, transmitted or received by a surface, per unit solid angle per unit projected area. This is a directional quantity. This is sometimes also confusingly called "intensity". |
Spectral radiance Specific intensity |
Le,Ω,ν[nb 3] | watt per steradian per square metre per hertz | W⋅sr−1⋅m−2⋅Hz−1 | M⋅T−2 | Radiance of a surface per unit frequency or wavelength. The latter is commonly measured in W⋅sr−1⋅m−2⋅nm−1. This is a directional quantity. This is sometimes also confusingly called "spectral intensity". |
Le,Ω,λ[nb 4] | watt per steradian per square metre, per metre | W⋅sr−1⋅m−3 | M⋅L−1⋅T−3 | ||
Irradiance Flux density |
Ee[nb 2] | watt per square metre | W/m2 | M⋅T−3 | Radiant flux received by a surface per unit area. This is sometimes also confusingly called "intensity". |
Spectral irradiance Spectral flux density |
Ee,ν[nb 3] | watt per square metre per hertz | W⋅m−2⋅Hz−1 | M⋅T−2 | Irradiance of a surface per unit frequency or wavelength. This is sometimes also confusingly called "spectral intensity". Non-SI units of spectral flux density include jansky (1 Jy = 10−26 W⋅m−2⋅Hz−1) and solar flux unit (1 sfu = 10−22 W⋅m−2⋅Hz−1 = 104 Jy). |
Ee,λ[nb 4] | watt per square metre, per metre | W/m3 | M⋅L−1⋅T−3 | ||
Radiosity | Je[nb 2] | watt per square metre | W/m2 | M⋅T−3 | Radiant flux leaving (emitted, reflected and transmitted by) a surface per unit area. This is sometimes also confusingly called "intensity". |
Spectral radiosity | Je,ν[nb 3] | watt per square metre per hertz | W⋅m−2⋅Hz−1 | M⋅T−2 | Radiosity of a surface per unit frequency or wavelength. The latter is commonly measured in W⋅m−2⋅nm−1. This is sometimes also confusingly called "spectral intensity". |
Je,λ[nb 4] | watt per square metre, per metre | W/m3 | M⋅L−1⋅T−3 | ||
Radiant exitance | Me[nb 2] | watt per square metre | W/m2 | M⋅T−3 | Radiant flux emitted by a surface per unit area. This is the emitted component of radiosity. "Radiant emittance" is an old term for this quantity. This is sometimes also confusingly called "intensity". |
Spectral exitance | Me,ν[nb 3] | watt per square metre per hertz | W⋅m−2⋅Hz−1 | M⋅T−2 | Radiant exitance of a surface per unit frequency or wavelength. The latter is commonly measured in W⋅m−2⋅nm−1. "Spectral emittance" is an old term for this quantity. This is sometimes also confusingly called "spectral intensity". |
Me,λ[nb 4] | watt per square metre, per metre | W/m3 | M⋅L−1⋅T−3 | ||
Radiant exposure | He | joule per square metre | J/m2 | M⋅T−2 | Radiant energy received by a surface per unit area, or equivalently irradiance of a surface integrated over time of irradiation. This is sometimes also called "radiant fluence". |
Spectral exposure | He,ν[nb 3] | joule per square metre per hertz | J⋅m−2⋅Hz−1 | M⋅T−1 | Radiant exposure of a surface per unit frequency or wavelength. The latter is commonly measured in J⋅m−2⋅nm−1. This is sometimes also called "spectral fluence". |
He,λ[nb 4] | joule per square metre, per metre | J/m3 | M⋅L−1⋅T−2 | ||
See also: |
- ^ Standards organizations recommend that radiometric quantities should be denoted with suffix "e" (for "energetic") to avoid confusion with photometric or photon quantities.
- ^ a b c d e Alternative symbols sometimes seen: W or E for radiant energy, P or F for radiant flux, I for irradiance, W for radiant exitance.
- ^ a b c d e f g Spectral quantities given per unit frequency are denoted with suffix "ν" (Greek letter nu, not to be confused with a letter "v", indicating a photometric quantity.)
- ^ a b c d e f g Spectral quantities given per unit wavelength are denoted with suffix "λ".
- ^ a b Directional quantities are denoted with suffix "Ω".
Light can also be characterised by:
- amplitude,
- color, wavelength, or frequency, and
- polarization (or angle of vibration).
Light sources

There are many sources of light. The most common light sources are thermal: a body at a given temperature emits a characteristic spectrum of black body radiation. Examples include sunlight (the radiation emitted by the chromosphere of the Sun at around 6,000 K peaks in the visible region of the electromagnetic spectrum), incandescent light bulbs (which emit only around 10% of their energy as visible light and the remainder as infrared), and glowing solid particles in flames. The peak of the blackbody spectrum is in the infrared for relatively cool objects like human beings. As the temperature increases, the peak shifts to shorter wavelengths, producing first a red glow, then a white one, and finally a blue color as the peak moves out of the visible part of the spectrum and into the ultraviolet. These colors can be seen when metal is heated to "red hot" or "white hot". The blue color is most commonly seen in a gas flame or a welder's torch.
Atoms emit and absorb light at characteristic energies. This produces "emission lines" in the spectrum of each atom. Emission can be spontaneous, as in light-emitting diodes, gas discharge lamps (such as neon lamps and neon signs, mercury-vapor lamps, etc.), and flames (light from the hot gas itself—so, for example, sodium in a gas flame emits characteristic yellow light). Emission can also be stimulated, as in a laser or a microwave maser.
Acceleration of a free charged particle, such as an electron, can produce visible radiation: cyclotron radiation, synchrotron radiation, and bremsstrahlung radiation are all examples of this. Particles moving through a medium faster than the speed of light in that medium can produce visible Cherenkov radiation.
Certain chemicals produce visible radiation by chemoluminescence. In living things, this process is called bioluminescence. For example, fireflies produce light by this means, and boats moving through water can disturb plankton which produce a glowing wake.
Certain substances produce light when they are illuminated by more energetic radiation, a process known as fluorescence. This is used in fluorescent lights. Some substances emit light slowly after excitation by more energetic radiation. This is known as phosphorescence.
Phosphorescent materials can also be excited by bombarding them with subatomic particles. Cathodoluminescence is one example of this. This mechanism is used in cathode ray tube televisions.
Certain other mechanisms can produce light:
- scintillation
- electroluminescence
- sonoluminescence
- triboluminescence
- radioactive decay
- particle-antiparticle annihilation
Theories about light
Indian theories
In ancient India, the philosophical schools of Samkhya and Vaisheshika, from around the 6th–5th century BC, developed theories on light. According to the Samkhya school, light is one of the five fundamental "subtle" elements (tanmatra) out of which emerge the gross elements. The atomicity of these elements is not specifically mentioned and it appears that they were actually taken to be continuous.
On the other hand, the Vaisheshika school gives an atomic theory of the physical world on the non-atomic ground of ether, space and time. (See Indian atomism.) The basic atoms are those of earth (prthivı), water (apas), fire (tejas), and air (vayu), that should not be confused with the ordinary meaning of these terms. These atoms are taken to form binary molecules that combine further to form larger molecules. Motion is defined in terms of the movement of the physical atoms and it appears that it is taken to be non-instantaneous. Light rays are taken to be a stream of high velocity of tejas (fire) atoms. The particles of light can exhibit different characteristics depending on the speed and the arrangements of the tejas atoms.
Later in 499 CE, Aryabhata, who proposed a heliocentric solar system of gravitation in his Aryabhatiya, wrote that the planets and the Moon do not have their own light but reflect the light of the Sun.
The Indian Buddhists, such as Dignāga in the 5th century and Dharmakirti in the 7th century, developed a type of atomism that is a philosophy about reality being composed of atomic entities that are momentary flashes of light or energy. They viewed light as being an atomic entity equivalent to energy, similar to the modern concept of photons, though they also viewed all matter as being composed of these light/energy particles.
Hellenistic theories
In 55 BC, Lucretius, continuing the ideas of earlier atomists, wrote that light and heat from the Sun were composed of minute particles.
Ptolemy (c. 2nd century CE) wrote about the refraction of light, and developed a theory of vision that objects are seen by rays of light emanating from the eyes.
Optical theory
The Persian scientist Alhazen Abu Ali al-Hasan ibn al-Haytham (c. 965-1040), also known as Alhazen, developed a broad theory that explained vision, using geometry and anatomy, which stated that each point on an illuminated area or object radiates light rays in every direction, but that only one ray from each point, which strikes the eye perpendicularly, can be seen. The other rays strike at different angles and are not seen. He used the example of the pinhole camera, which produces an inverted image, to support his argument. This contradicted Ptolemy's theory of vision that objects are seen by rays of light emanating from the eyes. Alhazen held light rays to be streams of minute particles that travelled at a finite speed. He improved Ptolemy's theory of the refraction of light, and went on to discover the laws of refraction.
He also carried out the first experiments on the dispersion of light into its constituent colors. His major work Kitab-at-Manazir was translated into Latin in the Middle Ages, as well his book dealing with the colors of sunset. He dealt at length with the theory of various physical phenomena like shadows, eclipses, the rainbow. He also attempted to explain binocular vision, and gave a correct explanation of the apparent increase in size of the sun and the moon when near the horizon. Through these extensive researches on optics, is considered as the father of modern optics.
The 'plenum'
René Descartes (1596-1650) held that light was a disturbance of the plenum, the continuous substance of which the universe was composed. In 1637 he published a theory of the refraction of light which wrongly assumed that light travelled faster in a denser medium, by analogy with the behaviour of sound waves. Descartes' theory is often regarded as the forerunner of the wave theory of light.
Particle theory
Pierre Gassendi (1592-1655), an atomist, proposed a particle theory of light which was published posthumously in the 1660s. Isaac Newton studied Gassendi's work at an early age, and preferred his view to Descartes' theory of the plenum. He stated in his Hypothesis of Light of 1675 that light was composed of corpuscles (particles of matter) which were emitted in all directions from a source. One of Newton's arguments against the wave nature of light was that waves were known to bend around obstacles, while light travelled only in straight lines. He did, however, explain the phenomenon of the diffraction of light (which had been observed by Francesco Grimaldi) by allowing that a light particle could create a localised wave in the aether.
Newton's theory could be used to predict the reflection of light, but could only explain refraction by incorrectly assuming that light accelerated upon entering a denser medium because the gravitational pull was greater. Newton published the final version of his theory in his Opticks of 1704. His reputation helped the particle theory of light to dominate physics during the 18th century.
Wave theory
In the 1660s, Robert Hooke published a wave theory of light. Christian Huygens worked out his own wave theory of light in 1678, and published it in his Treatise on light in 1690. He proposed that light was emitted in all directions as a series of waves in a medium called the aether. As waves are not affected by gravity, it was assumed that they slowed down upon entering a denser medium.
The wave theory predicted that light waves could interfere with each other like sound waves (as noted in the 18th century by Thomas Young), and that light could be polarized. Young showed by means of a diffraction experiment that light behaved as waves. He also proposed that different colors were caused by different wavelengths of light, and explained color vision in terms of three-colored receptors in the eye.
Another supporter of the wave theory was Euler. He argued in Nova theoria lucis et colorum (1746) that diffraction could more easily be explained by a wave theory.
Later, Fresnel independently worked out his own wave theory of light, and presented it to the Académie des Sciences in 1817. Simeon Denis Poisson added to Fresnel's mathematical work to produce a convincing argument in favour of the wave theory, helping to overturn Newton's corpuscular theory.
The weakness of the wave theory was that light waves, like sound waves, would need a medium for transmission. A hypothetical substance called the luminiferous aether was proposed, but its existence was cast into strong doubt by the Michelson-Morley experiment.
Newton's corpuscular theory implied that light would travel faster in a denser medium, while the wave theory of Huygens and others implied the opposite. At that time, the speed of light could not be measured accurately enough to decide which theory was correct. The first to make a sufficiently accurate measurement was Léon Foucault, in 1850. His result supported the wave theory, and the classical particle theory was finally abandoned.
Electromagnetic theory
In 1845, Faraday discovered that the angle of polarisation of a beam of light as it passed through a polarising material could be altered by a magnetic field, an effect now known as Faraday rotation. This was the first evidence that light was related to electromagnetism. Faraday proposed in 1847 that light was a high-frequency electromagnetic vibration, which could propagate even in the absence of a medium such as the aether.
Faraday's work inspired James Clerk Maxwell to study electromagnetic radiation and light. Maxwell discovered that self-propagating electromagnetic waves would travel through space at a constant speed, which happened to be equal to the previously measured speed of light. From this, Maxwell concluded that light was a form of electromagnetic radiation: he first stated this result in 1862 in On Physical Lines of Force. In 1873, he published A Treatise on Electricity and Magnetism, which contained a full mathematical description of the behaviour of electric and magnetic fields, still known as Maxwell's equations. The technology of radio transmission was, and still is, based on this theory.
The constant speed of light predicted by Maxwell's equations contradicted the mechanical laws of motion that had been unchallenged since the time of Galileo, which stated that all speeds were relative to the speed of the observer. A solution to this contradiction would later be found by Albert Einstein.
Particle theory revisited
The wave theory was accepted until the late 19th century, when Einstein described the photoelectric effect, by which light striking a surface caused electrons to change their momentum, which indicated a particle-like nature of light. This clearly contradicted the wave theory, and for years physicists tried in vain to resolve this contradiction.
Quantum theory
In 1900, Max Planck described quantum theory, in which light is considered to be as a particle that could exist in discrete amounts of energy only. These packets were called quanta, and the particle of light was given the name photon, to correspond with other particles being described around this time, such as the electron and proton. A photon has an energy, E, proportional to its frequency, f, by
where h is Planck's constant, is the wavelength and c is the speed of light.
As it originally stood, this theory did not explain the simultaneous wave-like nature of light, though Planck would later work on theories that did. The Nobel Committee awarded Planck the Physics Prize in 1918 for his part in the founding of quantum theory.
Wave-particle duality
The modern theory that explains the nature of light is wave-particle duality, described by Albert Einstein in the early 1900s, based on his work on the photoelectric effect and Planck's results. Einstein determined that the energy of a photon is proportional to its frequency. More generally, the theory states that everything has both a particle nature and a wave nature, and various experiments can be done to bring out one or the other. The particle nature is more easily discerned if an object has a large mass, so it took until an experiment by Louis de Broglie in 1924 to realise that electrons also exhibited wave-particle duality. Einstein received the Nobel Prize in 1921 for his work with the wave-particle duality on photons, and de Broglie followed in 1929 for his extension to other particles.
A light wave
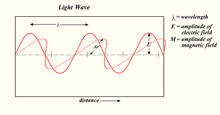
The electric and magnetic fields are perpendicular to the direction of travel and to each other. This picture depicts a very special case, linearly polarized light. See Polarization for a description of the general case and an explanation of linear polarization.
While these relations of the electric and magnetic fields are always true, the subtle difference in the general case is that the direction and amplitude of the magnetic (or electric) field can vary, in one place, with time, or, in one instant, can vary along the direction of propagation.
References
- M. Muller. Rig-Veda-Samhita together with the Commentary of Sayana, Oxford University Press, London, 1890.
- B. K. Matilal. Nyaya-Vaisesika, Otto Harrassowitz, Wiesbaden, 1977.
- K. H. Potter, Indian Metaphysics and Epistemology, Princeton University Press, Princeton, 1977.
- G. J. Larson and R. S. Bhattacharya. Samkhya: A Dualist Tradition in Indian Philosophy, Princeton University Press, Princeton, 1987.
- S. S. De. In Issues in Vedic Astronomy and Astrology, Motilal Banarsidass, 1992.
- P. V. Vartak. Scientific Knowledge in the Vedas, Nag Publishers, 1995.
- S. Kak. "The Speed of Light and Purāṇic Cosmology". In T. R. N. Rao and S. Kak, Computing Science in Ancient India, pages 80–90. USL Press, Lafayette, 1998. Available as e-print physics/9804020 on the arXiv.
See also

- Color temperature
- Huygens' principle
- Fermat's principle
- International Commission on Illumination
- Light beam - in particular about light beams visible from the side
- Light pollution
- Lighting
- Photic sneeze reflex
- Photometry
- Spectrometry
- The Science of Spectroscopy - supported by NASA. Spectroscopy education wiki and films - introduction to light, its uses in NASA, space science, astronomy, medicine & health, environmental research, and consumer products.